Engineering:475 °C embrittlement
Duplex stainless steels are a family of alloys with a two-phase microstructure consisting of both austenitic (face-centred cubic) and ferritic (body-centred cubic) phases. They offer excellent mechanical properties, corrosion resistance, and toughness compared to other types of stainless steel. However, duplex stainless steel can be susceptible to a phenomenon known as 475 °C embrittlement or duplex stainless steel age hardening, which is a type of aging process that causes loss of plasticity in duplex stainless steel when it is heated in the range of 250 to 550 °C (480 to 1,020 °F). At this temperature range, spontaneous phase separation of the ferrite phase into iron-rich and chromium-rich nanophases occurs, with no change in the mechanical properties of the austenite phase. This type of embrittlement is due to precipitation hardening, which makes the material become brittle and prone to cracking.
Duplex stainless steel
- stainless steel that has a two-phase microstructure consisting of both austenitic (face-centred cubic) and ferritic (body-centred cubic) phases.[3][4] This dual-phase structure gives duplex stainless steel a combination of mechanical and corrosion-resistant properties that are superior to those of either austenitic or ferritic stainless steel alone.[3][4] The austenitic phase provides the steel with good ductility, high toughness, and high corrosion resistance, especially in acidic and chloride-containing environments.[3][4] The ferritic phase, on the other hand, provides the steel with good strength, high resistance to stress corrosion cracking, and high resistance to pitting and crevice corrosion.[3][4] They are therefore used extensively in the offshore oil and gas industry for pipework systems, manifolds, risers, etc. and in the petrochemical industry in the form of pipelines and pressure vessels.[3] Duplex stainless steel is a type of
A duplex stainless steel mixture of austenite and ferrite microstructure is not necessarily in equal proportions, and where the alloy solidifies as ferrite, it is partially transformed to austenite when the temperature falls to around 1,000 °C (1,830 °F).[5][6] Duplex steels have a higher chromium content compared to austenitic stainless steel, 20–28%; higher molybdenum, up to 5%; lower nickel, up to 9%; and 0.05–0.50% nitrogen.[6][5] Thus, duplex stainless steel alloys have good corrosion resistance and higher strength than standard austenitic stainless steels such as type 304 or 316.[7][4]
Alpha (α) phase is a ferritic phase with body-centred cubic (BCC) structure, Im[math]\displaystyle{ \bar{3} }[/math]m [229] space group, 2.866 Å lattice parameter, and has one twinning system {112}<111> and three slip systems {110}<111>, {112}<111> and {123}<111>; however, the last system rarely activates.[8][9] Gamma ([math]\displaystyle{ \gamma }[/math]) phase is austenitic with a face-centred cubic (FCC) structure, Fm[math]\displaystyle{ \bar{3} }[/math]m [259] space group, and 3.66 Å lattice parameter. It normally has more nickel, copper, and interstitial carbon and nitrogen.[10] Plastic deformation occurs in austenite more readily than in ferrite.[11][2] During deformation, straight slip bands form in the austenite grains and propagate to the ferrite-austenite grain boundaries, assisting in the slipping of the ferrite phase. Curved slip bands also form due to the bulk-ferrite-grain deformation.[12][13][14] The formation of slip bands indicates a concentrated unidirectional slip on certain planes causing a stress concentration.[15]
Age hardening by spinodal decomposition
Duplex stainless steel can have limited toughness due to its large ferritic grain size, and its tendencies to hardening and embrittlement, i.e., loss of plasticity, at temperatures ranging from 250 to 550 °C (482 to 1,022 °F), especially at 475 °C (887 °F).[18] At this temperature range, spinodal decomposition of the supersaturated solid ferrite solution into iron-rich nanophase ([math]\displaystyle{ \acute{a} }[/math]) and chromium-rich nanophase ([math]\displaystyle{ \acute{a}\acute{} }[/math]), accompanied by G-phase precipitation, occurs.[18][19][20] This makes the ferrite phase a preferential initiation site for micro-cracks.[21] This is because aging encourages Σ3 {112}<111> ferrite deformation twinning at slow strain rate and room temperature in tensile or compressive deformation, nucleating from local stress concentration sites,[18][22] and parent-twinning boundaries, with 60° (in or out) misorientation, are suitable for cleavage crack nucleation.[22][23][24]
Spinodal decomposition refers to the spontaneous separation of a phase into two coherent phases via uphill diffusion, i.e., from a region of lower concentration to a region of higher concentration resulting in a negative diffusion coefficient [math]\displaystyle{ {d^2G \over dx^2} }[/math], without a barrier to nucleation due to the phase being thermodynamically unstable (i.e., miscibility gap, [math]\displaystyle{ \acute{a} }[/math] + [math]\displaystyle{ \acute{a}\acute{} }[/math] region in the figure),[25] where [math]\displaystyle{ G }[/math] is the Gibbs free energy per mole of solution and the composition. It increases hardness and decreases magneticity.[26] Miscibility gap describes the region in a phase diagram below the melting point of each compound where the solid phase splits into the liquid of two separated stable phases.[27]
For 475 °C embrittlement to occur, the chromium content needs to exceed 12%.[28] The addition of nickel accelerates the spinodal decomposition by promoting the iron-rich nanophase formation.[29] Nitrogen changes the distribution of chromium, nickel, and molybdenum in the ferrite phase but does not prevent the phase decomposition.[30] Other elements like molybdenum, manganese, and silicon do not affect the formation of iron-rich nanophase.[31] However, manganese and molybdenum partition to the iron-rich nanophase, while nickel partitions to the chromium-rich nanophase.[19]
Microscopy characterisation
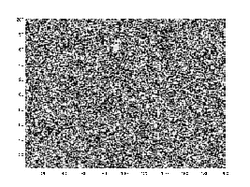
Using Field Emission Gun Transmission Electron Microscope FEG-TEM, the nanometre-scaled modulated structure of the decomposed ferrite was revealed as chromium-rich nanophase gave the bright image, and iron-rich darker image.[19] It also revealed that these modulated nanophases grow coarser with aging time.[19][32] Decomposed phases start as irregular rounded shapes with no particular arrangement, but with time the chromium-rich nanophase takes a plat shape aligned in the <110> directions.[32]
Consequences
Spinodal decomposition increases the hardening of the material due to the misfit between the chromium-rich and iron-rich nano-phases, internal stress, and variation of elastic modulus. The formation of coherent precipitates induces an equal but opposite strain, raising the system's free energy depending on the precipitate shape and matrix and precipitate elastic properties.[27][33] Around a spherical inclusion, the distortion is purely hydrostatic.[27]
G-phase precipitates appear prominently at grain boundaries.[20] and are phase rich in nickel, titanium, and silicon,[20] but chromium and manganese may substitute titanium sites.[34] G-phase precipitates occur during long-term aging, are encouraged by increasing nickel content in the ferrite phase,[34] and reduce corrosion resistance significantly.[35] It has ellipsoid morphology, FCC structure (Fm[math]\displaystyle{ \bar{3} }[/math]m), and 11.4 Å lattice parameter,[36] with a diameter less than 50 nm that increases with aging.[37][38]
Thus, the embrittlement is caused by dislocations impediment/ locking by the spinodally decomposed matrix[39][40] and strain around G-phase precipitates,[41] i.e., internal stress relaxation by the formation of Cottrell atmosphere.[42]
Furthermore, the ferrite hardness increases with aging time, the hardness of the ductile austenite phase remains nearly unchanged[39][40][43] due to faster diffusivity in ferrite compared to the austenite.[26] However, austenite undergoes a substitutional redistribution of elements, enhancing galvanic corrosion between the two phases.[44]
Treatment
550 °C heat treatment can reverse spinodal decomposition but not affect the G-phase precipitates.[45] The ferrite matrix spinodal decomposition can be substantially reversed by introducing an external pulsed electric current that changes the system's free energy due to the difference in electrical conductivity between the nanophases and the dissolution of G-phase precipitates.[46][47]
Cyclic loading suppresses spinodal decomposition,[48] and radiation accelerates it but changes the decomposition nature from an interconnected network of modulated nanophases to isolated islands.[49]
References
- ↑ 1.0 1.1 1.2 Mohamed Koko, A. (2022). In situ full-field characterisation of strain concentrations (deformation twins, slip bands and cracks) (PhD Thesis thesis). University of Oxford. Archived from the original on 2023-02-01. Retrieved 2023-03-02.
This article incorporates text from this source, which is available under the CC BY 4.0 license.
- ↑ 2.0 2.1 Koko, Abdalrhaman; Elmukashfi, Elsiddig; Becker, Thorsten H.; Karamched, Phani S.; Wilkinson, Angus J.; Marrow, T. James (2022-10-15). "In situ characterisation of the strain fields of intragranular slip bands in ferrite by high-resolution electron backscatter diffraction" (in en). Acta Materialia 239: 118284. doi:10.1016/j.actamat.2022.118284. ISSN 1359-6454. Bibcode: 2022AcMat.23918284K.
- ↑ 3.0 3.1 3.2 3.3 3.4 International Stainless Steel Forum (2020). "Duplex Stainless Steels". http://www.worldstainless.org/Files/issf/non-image-files/PDF/ISSF_Duplex_Stainless_Steels.pdf.
- ↑ 4.0 4.1 4.2 4.3 4.4 Peckner, Donald; Bernstein, I.M. (1977). "chapter 8". Handbook of Stainless Steels. McGraw Hill. ISBN 9780070491472.
- ↑ 5.0 5.1 Charles, Jacques (2010). Proceedings of the Duplex Stainless Steel Conference, Beaune (2010). EDP Sciences, Paris. pp. 29–82. https://pubs.kci-webshop.com/Webshop/Product/BOOKS/Duplex-Stainless-Steel---DSS-2010-Conference-Proceedings.html. Retrieved 2023-03-25.
- ↑ 6.0 6.1 Practical Guidelines for the Fabrication of Duplex Stainless Steels (3rd ed.). International Molybdenum Association (IMOA). 2014. ISBN 978-1-907470-09-7. http://www.imoa.info/download_files/stainless-steel/Duplex_Stainless_Steel_3rd_Edition.pdf. Retrieved 2023-03-25.
- ↑ Alvarez-Armas, Iris (2008). "Duplex Stainless Steels: Brief History and Some Recent Alloys" (in en). Recent Patents on Mechanical Engineering 1 (1): 51–57. doi:10.2174/2212797610801010051. https://www.eurekaselect.com/article/40020. Retrieved 2022-10-14.
- ↑ Du, C.; Maresca, F.; Geers, M. G. D.; Hoefnagels, J. P. M. (2018-03-01). "Ferrite slip system activation investigated by uniaxial micro-tensile tests and simulations" (in en). Acta Materialia 146: 314–327. doi:10.1016/j.actamat.2017.12.054. ISSN 1359-6454. Bibcode: 2018AcMat.146..314D. https://www.sciencedirect.com/science/article/pii/S1359645418300107.
- ↑ Koko, Abdalrhaman; Elmukashfi, Elsiddig; Dragnevski, Kalin; Wilkinson, Angus J.; Marrow, Thomas James (2021-10-01). "J-integral analysis of the elastic strain fields of ferrite deformation twins using electron backscatter diffraction" (in en). Acta Materialia 218: 117203. doi:10.1016/j.actamat.2021.117203. ISSN 1359-6454. Bibcode: 2021AcMat.21817203K. https://www.sciencedirect.com/science/article/pii/S1359645421005838.
- ↑ Nilsson, J.-O. (1992-08-01). "Super duplex stainless steels". Materials Science and Technology 8 (8): 685–700. doi:10.1179/mst.1992.8.8.685. ISSN 0267-0836. Bibcode: 1992MatST...8..685N. https://doi.org/10.1179/mst.1992.8.8.685. Retrieved 2022-10-14.
- ↑ Zieliński, W.; Świątnicki, W.; Barstch, M.; Messerschmidt, U. (2003-08-28). "Non-uniform distribution of plastic strain in duplex steel during TEM in situ deformation" (in en). Materials Chemistry and Physics 81 (2): 476–479. doi:10.1016/S0254-0584(03)00059-2. ISSN 0254-0584. https://www.sciencedirect.com/science/article/pii/S0254058403000592.
- ↑ Serre, I.; Salazar, D.; Vogt, J.-B. (September 2008). "Atomic force microscopy investigation of surface relief in individual phases of deformed duplex stainless steel" (in en). Materials Science and Engineering: A 492 (1–2): 428–433. doi:10.1016/j.msea.2008.04.060. https://linkinghub.elsevier.com/retrieve/pii/S0921509308005157. Retrieved 2022-10-14.
- ↑ "Microstructural Evolution and Mechanical Behavior of Thermally Aged Cast Duplex Stainless Steel". Materials 13 (24): 5636. December 2020. doi:10.3390/ma13245636. PMID 33321825. Bibcode: 2020Mate...13.5636L.
- ↑ Zhang, Qingdong; Ma, Sida; Jing, Tao (March 2019). "Mechanical Properties of a Thermally-aged Cast Duplex Stainless Steel by in Situ Tensile Test at the Service Temperature" (in en). Metals 9 (3): 317. doi:10.3390/met9030317. ISSN 2075-4701.
- ↑ Sangid, Michael D. (2013-12-01). "The physics of fatigue crack initiation" (in en). International Journal of Fatigue. Fatigue and Microstructure: A special issue on recent advances 57: 58–72. doi:10.1016/j.ijfatigue.2012.10.009. ISSN 0142-1123. https://www.sciencedirect.com/science/article/pii/S0142112312002940.
- ↑ Fan, Y.; Liu, T.G.; Xin, L.; Han, Y.M.; Lu, Y.H.; Shoji, T. (February 2021). "Thermal aging behaviors of duplex stainless steels used in nuclear power plant: A review" (in en). Journal of Nuclear Materials 544: 152693. doi:10.1016/j.jnucmat.2020.152693. Bibcode: 2021JNuM..54452693F. https://linkinghub.elsevier.com/retrieve/pii/S0022311520313015. Retrieved 2022-10-14.
- ↑ Bonny, G.; Terentyev, D.; Malerba, L. (October 2010). "New Contribution to the Thermodynamics of Fe-Cr Alloys as Base for Ferritic Steels" (in en). Journal of Phase Equilibria and Diffusion 31 (5): 439–444. doi:10.1007/s11669-010-9782-9. ISSN 1547-7037. http://link.springer.com/10.1007/s11669-010-9782-9. Retrieved 2022-10-14.
- ↑ 18.0 18.1 18.2 Örnek, Cem; Burke, M. G.; Hashimoto, T.; Engelberg, D. L. (April 2017). "748 K (475 °C) Embrittlement of Duplex Stainless Steel: Effect on Microstructure and Fracture Behavior" (in en). Metallurgical and Materials Transactions A 48 (4): 1653–1665. doi:10.1007/s11661-016-3944-2. ISSN 1073-5623. Bibcode: 2017MMTA...48.1653O.
- ↑ 19.0 19.1 19.2 19.3 Weng, K. L; Chen, H. R; Yang, J. R (2004-08-15). "The low-temperature aging embrittlement in a 2205 duplex stainless steel" (in en). Materials Science and Engineering: A 379 (1): 119–132. doi:10.1016/j.msea.2003.12.051. ISSN 0921-5093. https://www.sciencedirect.com/science/article/pii/S0921509304000590.
- ↑ 20.0 20.1 20.2 Beattie, H. J.; Versnyder, F. L. (July 1956). "A New Complex Phase in a High-Temperature Alloy" (in en). Nature 178 (4526): 208–209. doi:10.1038/178208b0. ISSN 1476-4687. Bibcode: 1956Natur.178..208B. https://www.nature.com/articles/178208b0. Retrieved 2022-10-14.
- ↑ Liu, Gang; Li, Shi-Lei; Zhang, Hai-Long; Wang, Xi-Tao; Wang, Yan-Li (August 2018). "Characterization of Impact Deformation Behavior of a Thermally Aged Duplex Stainless Steel by EBSD" (in en). Acta Metallurgica Sinica (English Letters) 31 (8): 798–806. doi:10.1007/s40195-018-0708-6. ISSN 1006-7191.
- ↑ 22.0 22.1 Marrow, T. J.; Humphreys, A. O.; Strangwood, M. (July 1997). "The Crack Initiation Toughness for Brittle Fracture of Super Duplex Stainless Steel" (in en). Fatigue & Fracture of Engineering Materials & Structures 20 (7): 1005–1014. doi:10.1111/j.1460-2695.1997.tb01543.x. https://onlinelibrary.wiley.com/doi/10.1111/j.1460-2695.1997.tb01543.x. Retrieved 2022-10-14.
- ↑ Kato, Masaharu (January 1981). "Hardening by spinodally modulated structure in b.c.c. alloys" (in en). Acta Metallurgica 29 (1): 79–87. doi:10.1016/0001-6160(81)90088-2. https://linkinghub.elsevier.com/retrieve/pii/0001616081900882. Retrieved 2022-10-14.
- ↑ Marrow, T. J.; Harris, C. (July 1996). "The Fracture Mechanism of 475°C Embrittlement in a Duplex Stainless Steel" (in en). Fatigue & Fracture of Engineering Materials & Structures 19 (7): 935–947. doi:10.1111/j.1460-2695.1996.tb01028.x. https://onlinelibrary.wiley.com/doi/10.1111/j.1460-2695.1996.tb01028.x. Retrieved 2022-10-14.
- ↑ Mburu, Sarah; Kolli, R. Prakash; Perea, Daniel E.; Schwarm, Samuel C.; Eaton, Arielle; Liu, Jia; Patel, Shiv; Bartrand, Jonah et al. (April 2017). "Effect of aging temperature on phase decomposition and mechanical properties in cast duplex stainless steels" (in en). Materials Science and Engineering: A 690: 365–377. doi:10.1016/j.msea.2017.03.011.
- ↑ 26.0 26.1 Örnek, C.; Burke, M. G.; Hashimoto, T.; Lim, J. J. H.; Engelberg, D. L. (2017-05-26). "475°C Embrittlement of Duplex Stainless Steel—A Comprehensive Microstructure Characterization Study" (in en). Materials Performance and Characterization 6 (3): MPC20160088. doi:10.1520/MPC20160088. http://www.astm.org/doiLink.cgi?MPC20160088. Retrieved 2022-10-14.
- ↑ 27.0 27.1 27.2 Porter, David A.; Easterling, Kenneth E.; Sherif, Mohamed Y. (2021). Phase Transformations in Metals and Alloys. [S.l.]: CRC Press. ISBN 978-1-000-46779-6. OCLC 1267751972. https://www.worldcat.org/oclc/1267751972.
- ↑ Sahu, J. K.; Krupp, U.; Ghosh, R. N.; Christ, H. -J. (2009-05-20). "Effect of 475°C embrittlement on the mechanical properties of duplex stainless steel" (in en). Materials Science and Engineering: A 508 (1): 1–14. doi:10.1016/j.msea.2009.01.039. ISSN 0921-5093. https://www.sciencedirect.com/science/article/pii/S0921509309000197.
- ↑ Miller, M. K.; Russell, K. F. (1996-03-02). "Comparison of the rate of decomposition in Fe45%Cr, Fe45%Cr5%Ni and duplex stainless steels" (in en). Applied Surface Science. Proceedings of the 42nd International Field Emission Symposium 94-95: 398–402. doi:10.1016/0169-4332(95)00403-3. ISSN 0169-4332. Bibcode: 1996ApSS...94..398M. https://dx.doi.org/10.1016/0169-4332%2895%2900403-3.
- ↑ Bliznuk, T.; Mola, M.; Polshin, E.; Pohl, M.; Gavriljuk, V. (2005-09-25). "Effect of nitrogen on short-range atomic order in the feritic δ phase of a duplex steel" (in en). Materials Science and Engineering: A 405 (1): 11–17. doi:10.1016/j.msea.2005.05.094. ISSN 0921-5093. https://www.sciencedirect.com/science/article/pii/S0921509305006210.
- ↑ Solomon, H. D.; Levinson, Lionel M. (1978-03-01). "Mössbauer effect study of '475°c embrittlement' of duplex and ferritic stainless steels" (in en). Acta Metallurgica 26 (3): 429–442. doi:10.1016/0001-6160(78)90169-4. ISSN 0001-6160. https://dx.doi.org/10.1016/0001-6160%2878%2990169-4.
- ↑ 32.0 32.1 Soriano-Vargas, Orlando; Avila-Davila, Erika O.; Lopez-Hirata, Victor M.; Cayetano-Castro, Nicolas; Gonzalez-Velazquez, Jorge L. (May 2010). "Effect of spinodal decomposition on the mechanical behavior of Fe–Cr alloys" (in en). Materials Science and Engineering: A 527 (12): 2910–2914. doi:10.1016/j.msea.2010.01.020. https://linkinghub.elsevier.com/retrieve/pii/S0921509310000353. Retrieved 2022-10-14.
- ↑ Cahn, John W (1961-09-01). "On spinodal decomposition" (in en). Acta Metallurgica 9 (9): 795–801. doi:10.1016/0001-6160(61)90182-1. ISSN 0001-6160. https://dx.doi.org/10.1016/0001-6160%2861%2990182-1. Retrieved 2022-10-14.
- ↑ 34.0 34.1 Sakata, Mikihiro; Kadoi, Kota; Inoue, Hiroshige (December 2021). "Acceleration of 475 °C embrittlement in weld metal of 22 mass% Cr-duplex stainless steel" (in en). Materials Today Communications 29: 102800. doi:10.1016/j.mtcomm.2021.102800.
- ↑ Xue, Fei; Shi, Fangjie; Zhang, Chuangju; Zheng, Qiaoling; Yi, Dawei; Li, Xiuqing; Li, Yefei (2021-07-21). "The Microstructure and Mechanical and Corrosion Behaviors of Thermally Aged Z3CN20-09M Cast Stainless Steel for Primary Coolant Pipes of Nuclear Power Plants" (in en). Coatings 11 (8): 870. doi:10.3390/coatings11080870. ISSN 2079-6412.
- ↑ Li, Shilei; Wang, Yanli; Wang, Xitao; Xue, Fei (September 2014). "G-phase precipitation in duplex stainless steels after long-term thermal aging: A high-resolution transmission electron microscopy study" (in en). Journal of Nuclear Materials 452 (1–3): 382–388. doi:10.1016/j.jnucmat.2014.05.069. Bibcode: 2014JNuM..452..382L. https://linkinghub.elsevier.com/retrieve/pii/S0022311514003468. Retrieved 2022-10-14.
- ↑ Hamaoka, T.; Nomoto, A.; Nishida, K.; Dohi, K.; Soneda, N. (December 2012). "Effects of aging temperature on G-phase precipitation and ferrite-phase decomposition in duplex stainless steel" (in en). Philosophical Magazine 92 (34): 4354–4375. doi:10.1080/14786435.2012.707340. ISSN 1478-6435. Bibcode: 2012PMag...92.4354H. http://www.tandfonline.com/doi/abs/10.1080/14786435.2012.707340. Retrieved 2022-10-14.
- ↑ Mateo, A; Llanes, L; Anglada, M; Redjaimia, A; Metauer, G (1997). "Characterization of the intermetallic G-phase in an AISI 329 duplex stainless steel". Journal of Materials Science 32 (17): 4533–4540. doi:10.1023/A:1018669217124. http://link.springer.com/10.1023/A:1018669217124. Retrieved 2022-10-14.
- ↑ 39.0 39.1 Zhang, Qingdong; Singaravelu, Arun Sundar S.; Zhao, Yongfeng; Jing, Tao; Chawla, Nikhilesh (2019-01-16). "Mechanical properties of a thermally-aged cast duplex stainless steel by nanoindentation and micropillar compression" (in en). Materials Science and Engineering: A 743: 520–528. doi:10.1016/j.msea.2018.11.112. ISSN 0921-5093. https://www.sciencedirect.com/science/article/pii/S0921509318316344.
- ↑ 40.0 40.1 Lee, Ho Jung; Kong, Byeong Seo; Obulan Subramanian, Gokul; Heo, Jaewon; Jang, Changheui; Lee, Kyoung-Soo (2018-10-01). "Evaluation of thermal aging of δ-ferrite in austenitic stainless steel weld using nanopillar compression test" (in en). Scripta Materialia 155: 32–36. doi:10.1016/j.scriptamat.2018.06.016. ISSN 1359-6462. https://www.sciencedirect.com/science/article/pii/S1359646218303701.
- ↑ Badyka, R.; Monnet, G.; Saillet, S.; Domain, C.; Pareige, C. (February 2019). "Quantification of hardening contribution of G-Phase precipitation and spinodal decomposition in aged duplex stainless steel: APT analysis and micro-hardness measurements" (in en). Journal of Nuclear Materials 514: 266–275. doi:10.1016/j.jnucmat.2018.12.002. Bibcode: 2019JNuM..514..266B. https://linkinghub.elsevier.com/retrieve/pii/S0022311518312194. Retrieved 2022-10-14.
- ↑ Calcagnotto, Marion; Adachi, Yoshitaka; Ponge, Dirk; Raabe, Dierk (January 2011). "Deformation and fracture mechanisms in fine- and ultrafine-grained ferrite/martensite dual-phase steels and the effect of aging" (in en). Acta Materialia 59 (2): 658–670. doi:10.1016/j.actamat.2010.10.002. Bibcode: 2011AcMat..59..658C. https://linkinghub.elsevier.com/retrieve/pii/S1359645410006488. Retrieved 2022-10-14.
- ↑ Godfrey, T. J.; Smith, G. D.W. (November 1986). "The Atom Probe Analysis of a Cast Duplex Stainless Steel". Le Journal de Physique Colloques 47 (C7): C7–217–C7-222. doi:10.1051/jphyscol:1986738. ISSN 0449-1947. http://www.edpsciences.org/10.1051/jphyscol:1986738. Retrieved 2022-10-14.
- ↑ May, J.E.; de Sousa, C.A.C.; Kuri, S.E. (July 2003). "Aspects of the anodic behaviour of duplex stainless steels aged for long periods at low temperatures" (in en). Corrosion Science 45 (7): 1395–1403. doi:10.1016/S0010-938X(02)00244-5. https://linkinghub.elsevier.com/retrieve/pii/S0010938X02002445. Retrieved 2022-10-14.
- ↑ Lee, Ho Jung; Kong, Byeong Seo; Obulan Subramanian, Gokul; Heo, Jaewon; Jang, Changheui; Lee, Kyoung-Soo (October 2018). "Evaluation of thermal aging of δ-ferrite in austenitic stainless steel weld using nanopillar compression test" (in en). Scripta Materialia 155: 32–36. doi:10.1016/j.scriptamat.2018.06.016. https://linkinghub.elsevier.com/retrieve/pii/S1359646218303701. Retrieved 2022-10-14.
- ↑ Liu, Xuebing; Zhang, Xinfang (August 2018). "An ultrafast performance regeneration of aged stainless steel by pulsed electric current" (in en). Scripta Materialia 153: 86–89. doi:10.1016/j.scriptamat.2018.05.004. https://linkinghub.elsevier.com/retrieve/pii/S1359646218302859. Retrieved 2022-10-14.
- ↑ Liu, Xuebing; Lu, Wenjun; Zhang, Xinfang (January 2020). "Reconstructing the decomposed ferrite phase to achieve toughness regeneration in a duplex stainless steel" (in en). Acta Materialia 183: 51–63. doi:10.1016/j.actamat.2019.11.008. Bibcode: 2020AcMat.183...51L. https://linkinghub.elsevier.com/retrieve/pii/S1359645419307335. Retrieved 2022-10-14.
- ↑ Akita, Masayuki; Kakiuchi, Toshifumi; Uematsu, Yoshihiko (2011). "Microstructural Changes of High-Chromium Ferritic Stainless Steel Subjected to Cyclic Loading in 475°C Embrittlement Region" (in en). Procedia Engineering 10: 100–105. doi:10.1016/j.proeng.2011.04.019.
- ↑ Miller, M.K.; Stoller, R.E.; Russell, K.F. (June 1996). "Effect of neutron-irradiation on the spinodal decomposition of Fe-32% Cr model alloy" (in en). Journal of Nuclear Materials 230 (3): 219–225. doi:10.1016/0022-3115(96)80017-1. Bibcode: 1996JNuM..230..219M. https://linkinghub.elsevier.com/retrieve/pii/0022311596800171. Retrieved 2022-10-14.
Further reading
- Sahu, J.K.; Krupp, U.; Ghosh, R.N.; Christ, H.-J. (20 May 2009). "Effect of 475 °C embrittlement on the mechanical properties of duplex stainless steel". Materials Science and Engineering: A 508 (1–2): 1–14. doi:10.1016/j.msea.2009.01.039. https://www.sciencedirect.com/science/article/pii/S0921509309000197.
- Bonny, G; Terentyev, D; Malerba, L (2010). "New Contribution to the Thermodynamics of Fe-Cr Alloys as Base for Ferritic Steels.". Journal of Phase Equilibria and Diffusion 31 (5): 439–444. doi:10.1007/S11669-010-9782-9. https://link.springer.com/article/10.1007/s11669-010-9782-9.
![]() | Original source: https://en.wikipedia.org/wiki/475 °C embrittlement.
Read more |