Biology:Developmental plasticity
Developmental plasticity is a general term referring to changes in neural connections during development as a result of environmental interactions as well as neural changes induced by learning.[1] Much like neuroplasticity, or brain plasticity, developmental plasticity is specific to the change in neurons and synaptic connections as a consequence of developmental processes. A child creates most of these connections from birth to early childhood. There are three primary methods by which this may occur as the brain develops,[citation needed] but critical periods determine when lasting changes may form. Developmental plasticity may also be used in place of the term phenotypic plasticity when an organism in an embryonic or larval stage can alter its phenotype based on environmental factors.[2] However, a main difference between the two is that phenotypic plasticity experienced during adulthood can be reversible, whereas traits that are considered developmentally plastic set foundations during early development that remain throughout the life of the organism.[3]
Mechanisms
File:Long-Term-Relationships-between-Synaptic-Tenacity-Synaptic-Remodeling-and-Network-Activity-pbio.1000136.s008.ogv During development, the central nervous system acquires information via endogenous or exogenous factors as well as learning experiences. In acquiring and storing such information, the plastic nature of the central nervous system allows for the adaptation of existing neural connections in order to accommodate new information and experiences, resulting in developmental plasticity. This form of plasticity that occurs during development is the result of three predominant mechanisms: synaptic and homeostatic plasticity, and learning.[citation needed]
Synaptic plasticity
The underlying principle of synaptic plasticity is that synapses undergo an activity-dependent and selective strengthening or weakening so that new information can be stored.[4][5] Synaptic plasticity depends on numerous factors including the threshold of the presynaptic stimulus in addition to the relative concentrations of neurotransmitter molecules. Synaptic plasticity has long been implicated for its role in memory storage and is thought to play a key role in learning.[6][5] However, during developmental periods, synaptic plasticity is of particular importance, as changes in the network of synaptic connections can ultimately lead to changes in developmental milestones. For instance, the initial overproduction of synapses during development is key to plasticity that occurs in the visual and auditory cortices.[7] In experiments conducted by Hubel and Wiesel, the visual cortex of kittens exhibits synaptic plasticity in the refinement of neural connections following visual inputs. Correspondingly, in the absence of such inputs during development, the visual field fails to develop properly and can lead to abnormal structures and behavior.[8] Furthermore, research suggests that this initial overproduction of synapses during developmental periods provides the foundation by which many synaptic connections can be formed, thus resulting in more synaptic plasticity. In the same way that synapses are abundant during development, there are also refining mechanisms that assist in the maturation of synapses in neural circuits. This regulatory process allows the strengthening of important or frequently used synaptic connections while reducing the amount of weak connections.[9]
Homeostatic plasticity
In order to maintain balance, homeostatic controls exist to regulate the overall activity of neural circuits, specifically by regulating the destabilizing effects of developmental and learning processes that result in changes of synaptic strength. Homeostatic plasticity also helps regulate prolonged excitatory responses, which lead to a reduction in all of a neuron's synaptic responses.[10] Numerous pathways have recently been associated with homeostatic plasticity, though there is still no clear molecular mechanism. Synaptic scaling is one method that serves as a type of autoregulation, as neurons can recognize their own firing rates and notice when there are alterations; calcium-dependent signals control the levels of glutamate receptors at synaptic sites in response. Homeostatic mechanisms may be local or network-wide.[11]
Learning
While synaptic plasticity is considered to be a by-product of learning, learning involves interaction with the environment to acquire the new information or behavior; synaptic plasticity merely represents the change in strength or configuration of neural circuits.[12] Learning is crucial, as there is considerable interaction with the environment, which is when the potential for acquiring new information is greatest. By depending largely upon selective experiences, neural connections are altered and strengthened in a manner that is unique to those experiences.[13] Experimentally, this can be seen when rats are raised in an environment that allows ample social interaction, resulting in increased brain weight and cortical thickness. In contrast, the inverse is seen following rearing in an environment devoid of interaction.[14] Also, learning plays a considerable role in the selective acquisition of information and is markedly demonstrated when children develop one language instead of another. Another example of such experience-dependent plasticity that is critical during development is the occurrence of imprinting. This occurs as a result of a young child or animal being exposed to a novel stimulus and rapidly implementing a certain behavior in response.[15]
Neural development
The formation of the nervous system is one of the most crucial events in the developing embryo. The differentiation of stem cell precursors into specialized neurons gives rise to the formation of synapses and neural circuits, which is key to the principle of plasticity.[16] During this pivotal point in development, consequent developmental processes like the differentiation and specialization of neurons are highly sensitive to exogenous and endogenous factors.[17] For example, in utero exposure to nicotine has been linked to adverse effects, such as severe physical and cognitive deficits, due to the impediment of the normal acetylcholine receptor activation. In a recent study, the connection between such nicotine exposure and prenatal development was assessed. It was determined that nicotine exposure in early development can have a lasting and encompassing effect on neuronal structures, underlying the behavioral and cognitive defects observed in exposed humans and animals. Additionally, when proper synaptic function is disrupted through nicotine exposure, the overall circuit may become less sensitive and responsive to stimuli, resulting in compensatory developmental plasticity.[18] It is for this reason that exposure to various environmental factors during developmental periods can cause profound effects on subsequent neural functioning.
Neural refinement and connectivity
Initial stages of neural development begin early on in the fetus with spontaneous firing of the developing neuron.[19] These early connections are weak and often overlap at the terminal ends of the arbors.[20] The young neurons have complete potential of changing morphology during a time span classified as the critical period to achieve strengthened and refined synaptic connections. It is during this time that damaged neuronal connections can become functionally recovered. Large alterations in length and location of these neurons can occur until synaptic circuitry is further defined.[21] Although organization of neural connections begins at the earliest stages of development, activity-driven refinement only begins at birth when the individual neurons can be recognized as separate entities and start to enhance in specificity.[22] The gradual pruning of the initially blurry axonal branching occurs via competitive and facilitative mechanisms, relying on electrical activity at the synapses; axons that fire independently of each other tend to compete for territory, whereas axons that synchronously fire mutually amplify connections.[23] Until this architecture has been established, retinal focus remains diffuse. Perpetuation of these newly formed connections or the lack thereof depends on maintenance of electrical activities at the synapses. Upon refinement, the elaborate connections narrow and strengthen to fire only in response to specific stimuli to optimize visual acuity.[24] These mechanisms can malfunction with the introduction of toxins, which bind to sodium channels and suppress action potentials and consequently electrical activity between synapses.[25]
Quantification of synaptic networks has primarily been through retinal wave detection using Ca2+ fluorescent indicators. Prior to birth, retinal waves are seen to originate as clusters that propagate through the refractory region. These assays have been shown to provide spatiotemporal data on the random bursts of action potentials produced in a refractory period.[26] Another assay recently developed to assess the depth of neuronal connections utilizes the trans-neuronal spread of rabies.[27] This method of tracing employs the migration of a neurotropic virus through tightly interconnected neurons and specific site labeling of distinct connections.[28] Patch-clamping experiments and calcium imaging are often conducted based on preliminary results from this assay in order to detect spontaneous neuronal activity.[29] A method for in vitro synaptic quantification has been developed that uses immunofluorescence to measure synaptic density in different cell cultures.[30]
Critical period
The concept of critical periods is a widely accepted and prominent theme in development, with strong implications for developmental plasticity. Critical periods establish a time frame in which the shaping of neural networks can be carried out. During these critical periods in development, plasticity occurs as a result of changes in the structure or function of developing neural circuits. Such critical periods can also be experience-dependent, in the instance of learning via new experiences, or can be independent of the environmental experience and rely on biological mechanisms including endogenous or exogenous factors.[31] Some of the most pervading examples of this can be seen through the development of the visual cortex in addition to the acquisition of language as a result of developmental plasticity during the critical period.[8][32] A lesser known example, however, remains the critical development of respiratory control during developmental periods. At birth, the development of respiratory control neural circuits is incomplete, requiring complex interactions from both the environment and intrinsic factors. Experimentally exposing two-week-old kittens and rats to hyperoxic conditions completely eliminates the carotid chemoreceptor response to hypoxia, resulting in respiratory impairment. This has remarkable clinical significance, as newborn infants are often supplemented with considerable amounts of oxygen, which could detrimentally affect the way in which neural circuits for respiratory control develop during the critical period. When stimuli appear or experiences occur outside of the critical period, any potential outcome is typically not long-lasting.[33]
Spontaneous network activity
Another lesser known element of developmental plasticity includes spontaneous bursts of action potentials in developing neural circuits, also referred to as spontaneous network activity. During the early development of neural connections, excitatory synapses undergo spontaneous activation, resulting in elevated intracellular calcium levels that signal the onset of numerous signaling cascades and developmental processes. For example, prior to birth, neural circuits in the retina undergo spontaneous network activity, which has been found to elicit the formation of retinogeniculate connections.[34] Developmental spontaneous network activity is also exhibited in the proper formation of neuromuscular circuits.[35] It is believed that spontaneous network activity establishes a scaffold for subsequent learning and information acquisition following the initial establishment of synaptic connections during development.
Phenotypic plasticity
Reaction norms
The norm of reaction, or reaction norm, is a pattern of phenotypic plasticity that describes how a single genotype can produce an array of different phenotypes in response to different environmental conditions.[2] Furthermore, a reaction norm can be a graphical representation of organismal variation in phenotype in response to numerous environmental circumstances. The graphical representation of reaction norms is commonly parabolic in shape, which represents the variation in plasticity across a population.[36] Additionally, reaction norms allow organisms to evaluate the need for various phenotypes in response to the magnitude of the environmental signal.[2]
Polyphenisms
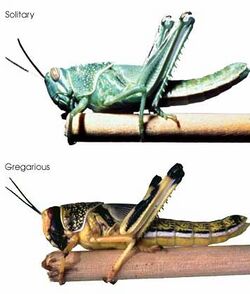
Polyphenism refers to the ability of a single genotype to produce a variety of phenotypes in response to different environmental conditions. In contrast to reaction norms, which produce a continuous range of phenotypes, polyphenisms allow a distinct phenotype to arise from altering environmental conditions.[37] An example of polyphenism can be seen in the Florida carpenter ant, Camponotus floridanus. For a developing ant embryo, a multitude of environmental signals can ultimately determine the adult ant morphology. For Florida carpenter ants, the end phenotype and behavior are determined by the morphology; developing ants can either become minor workers, major workers, or queen ants. Although the polyphenism of the ants has been documented, research is still needed to determine the molecular mechanisms for the induction of each unique phenotype.[38]
Environmental cues
Environmental cues in either the maternal or the embryonic environment can result in changes in the embryo. Embryonic development is a sensitive process and can be impacted by cues from predators,[39] light,[40] and/or temperature.[41] For example, in Daphnia, neonates exposed to predator cues displayed higher expression of genes related to digestion, reproductive function, and defense. It was hypothesized that this increase in gene expression would allow the Daphnia to defend themselves and that an increase in growth would result in a larger investment in future offspring. Subsequent generations exhibited a similar pattern, despite not being exposed to any predator cues, suggesting an inheritance of epigenetic expression factors.[39] An organism's sensitivity to light during development could be useful in predicting what phenotype may be the most beneficial in the future based on the foliage of the mature organism.[40] Several species, including alligators and tortoises, have temperature-dependent sex determination, where the sex of the organism is dependent on the environmental temperature during a crucial thermosensitive period. An active area of research involves the mechanisms of temperature sex determination, which have been hypothesized to be associated with the methylation of specific genes.[41]
See also
- Hebbian theory
- Long-term potentiation
- Long-term depression
- NMDA receptor
- GABA receptor
- Cultured neuronal network
- Developmental plasticity in Drosophila melanogaster
References
- ↑ Kolb, Bryan; Gibb, Robbin (2011). "Brain plasticity and behaviour in the developing brain". Journal of the Canadian Academy of Child and Adolescent Psychiatry 20 (4): 265–276. ISSN 2293-6122. PMID 22114608.
- ↑ 2.0 2.1 2.2 2.3 Gilbert, Scott F.; Epel, David (2015). Ecological developmental biology: the environmental regulation of development, health, and evolution (2nd ed.). Sunderland, Massachusetts, U.S.A.: Sinauer Associates, Inc. Publishers. ISBN 978-1-60535-344-9. OCLC 905089531.
- ↑ Lafuente, Elvira; Beldade, Patrícia (2019). "Genomics of Developmental Plasticity in Animals". Frontiers in Genetics 10. doi:10.3389/fgene.2019.00720. ISSN 1664-8021. PMID 31481970.
- ↑ "Neuromodulation, development and synaptic plasticity". Canadian Journal of Experimental Psychology 53 (1): 45–61. March 1999. doi:10.1037/h0087299. PMID 10389489.
- ↑ 5.0 5.1 Citri, Ami; Malenka, Robert C. (January 2008). "Synaptic Plasticity: Multiple Forms, Functions, and Mechanisms". Neuropsychopharmacology 33 (1): 18–41. doi:10.1038/sj.npp.1301559. ISSN 1740-634X. PMID 17728696.
- ↑ "How a child builds its brain: some lessons from animal studies of neural plasticity". Preventive Medicine 27 (2): 168–171. 1998. doi:10.1006/pmed.1998.0271. PMID 9578989.
- ↑ Phillips, Deborah; Shonkoff, Jack P. (2000). From neurons to neighborhoods: the science of early childhood development. Washington, DC: National Academies Press. ISBN 978-0-309-06988-5. OCLC 927036965. https://www.ncbi.nlm.nih.gov/books/NBK225562/.
- ↑ 8.0 8.1 Espinosa, J. Sebastian; Stryker, Michael P. (2012-07-26). "Development and Plasticity of the Primary Visual Cortex". Neuron 75 (2): 230–249. doi:10.1016/j.neuron.2012.06.009. PMID 22841309.
- ↑ Tau, Gregory Z.; Peterson, Bradley S. (January 2010). "Normal Development of Brain Circuits". Neuropsychopharmacology 35 (1): 147–168. doi:10.1038/npp.2009.115. ISSN 1740-634X. PMID 19794405.
- ↑ "Activity-dependent structural plasticity". Brain Research Reviews 60 (2): 287–305. May 2009. doi:10.1016/j.brainresrev.2008.12.023. PMID 19162072.
- ↑ Turrigiano, G. (2012-01-01). "Homeostatic Synaptic Plasticity: Local and Global Mechanisms for Stabilizing Neuronal Function". Cold Spring Harbor Perspectives in Biology 4 (1): a005736. doi:10.1101/cshperspect.a005736. ISSN 1943-0264. PMID 22086977.
- ↑ Kennedy, Mary B. (2013-12-30). "Synaptic Signaling in Learning and Memory". Cold Spring Harbor Perspectives in Biology 8 (2): a016824. doi:10.1101/cshperspect.a016824. ISSN 1943-0264. PMID 24379319.
- ↑ Fox, Sharon E.; Levitt, Pat; Nelson III, Charles A. (2010-01-01). "How the Timing and Quality of Early Experiences Influence the Development of Brain Architecture". Child Development 81 (1): 28–40. doi:10.1111/j.1467-8624.2009.01380.x. PMID 20331653.
- ↑ "Chemical and Anatomical Plasticity of Brain". Science 146 (3644): 610–619. October 1964. doi:10.1126/science.146.3644.610. PMID 14191699. Bibcode: 1964Sci...146..610B.
- ↑ Breed, Michael D.; Moore, Janice (2015). Animal behavior. Amsterdam: Academic Press. ISBN 978-0-12-801532-2. OCLC 943254906.
- ↑ Eric R. Kandel; James H. Schwartz; Thomas M. Jessell (2000). Principles of neural science (4th ed.). New York: McGraw-Hill, Health Professions Division. ISBN 0-8385-7701-6. OCLC 42073108.
- ↑ Archer, Trevor (2010-06-14). "Effects of Exogenous Agents on Brain Development: Stress, Abuse and Therapeutic Compounds: Effects of Exogenous Agents on Brain Development". CNS Neuroscience & Therapeutics 17 (5): 470–489. doi:10.1111/j.1755-5949.2010.00171.x. PMID 20553311.
- ↑ "Nicotine-induced plasticity during development: modulation of the cholinergic system and long-term consequences for circuits involved in attention and sensory processing". Neuropharmacology 56 (Suppl 1): 254–262. 2009. doi:10.1016/j.neuropharm.2008.07.020. PMID 18692078.
- ↑ Konkel, Lindsey (2018-11-20). "The Brain before Birth: Using fMRI to Explore the Secrets of Fetal Neurodevelopment". Environmental Health Perspectives 126 (11): 112001. doi:10.1289/ehp2268. ISSN 0091-6765.
- ↑ Grueber, W. B.; Sagasti, A. (2010-09-01). "Self-avoidance and Tiling: Mechanisms of Dendrite and Axon Spacing". Cold Spring Harbor Perspectives in Biology 2 (9): a001750. doi:10.1101/cshperspect.a001750. ISSN 1943-0264. PMID 20573716.
- ↑ Rice, Deborah; Barone Jr., Stan (2000-06-01). "Critical Periods of Vulnerability for the Developing Nervous System: Evidence from Humans and Animal Models". Environmental Health Perspectives 108 (3): 511–533. doi:10.1289/ehp.00108s3511. PMID 10852851.
- ↑ Tao, Huizhong W.; Poo, Mu-ming (2005-03-24). "Activity-Dependent Matching of Excitatory and Inhibitory Inputs during Refinement of Visual Receptive Fields". Neuron 45 (6): 829–836. doi:10.1016/j.neuron.2005.01.046. ISSN 0896-6273. PMID 15797545. https://www.cell.com/neuron/abstract/S0896-6273(05)00115-7.
- ↑ Cancedda, Laura; Poo, Mu-Ming (2009). "Synapse Formation and Elimination: Competition and the Role of Activity". in Binder, Marc D.; Hirokawa, Nobutaka; Windhorst, Uwe. Encyclopedia of Neuroscience. Berlin, Heidelberg: Springer. pp. 3932–3938. doi:10.1007/978-3-540-29678-2_5800. ISBN 978-3-540-23735-8.
- ↑ Demb, Jonathan B.; Singer, Joshua H. (2015-11-24). "Functional Circuitry of the Retina". Annual Review of Vision Science 1 (1): 263–289. doi:10.1146/annurev-vision-082114-035334. ISSN 2374-4642. PMID 28532365.
- ↑ Molecular Biology of the Cell. (4th ed.). Garland Science. 2002. https://www.ncbi.nlm.nih.gov/books/NBK26814/.
- ↑ Patel, Tapan P.; Man, Karen; Firestein, Bonnie L.; Meaney, David F. (2015-03-30). "Automated quantification of neuronal networks and single-cell calcium dynamics using calcium imaging". Journal of Neuroscience Methods 243: 26–38. doi:10.1016/j.jneumeth.2015.01.020. ISSN 0165-0270. PMID 25629800.
- ↑ "Modelling schizophrenia using human induced pluripotent stem cells". Nature 473 (7346): 221–225. May 2011. doi:10.1038/nature09915. PMID 21490598. Bibcode: 2011Natur.473..221B.
- ↑ "Rabies virus as a transneuronal tracer of neuronal connections". Advances in Virus Research 79: 165–202. 2011. doi:10.1016/B978-0-12-387040-7.00010-X. ISBN 9780123870407. PMID 21601048.
- ↑ "Patch-clamp recordings and calcium imaging followed by single-cell PCR reveal the developmental profile of 13 genes in iPSC-derived human neurons". Stem Cell Research 12 (1): 101–118. January 2014. doi:10.1016/j.scr.2013.09.014. PMID 24157591.
- ↑ Verstraelen, Peter; Garcia-Diaz Barriga, Gerardo; Verschuuren, Marlies; Asselbergh, Bob; Nuydens, Rony; Larsen, Peter H.; Timmermans, Jean-Pierre; De Vos, Winnok H. (2020-09-07). "Systematic Quantification of Synapses in Primary Neuronal Culture". iScience 23 (9): 101542. doi:10.1016/j.isci.2020.101542. ISSN 2589-0042. PMID 33083769.
- ↑ Cisneros-Franco, J. Miguel; Voss, Patrice; Thomas, Maryse E.; de Villers-Sidani, Etienne (2020), "Critical periods of brain development", Handbook of Clinical Neurology (Elsevier) 173: pp. 75–88, doi:10.1016/b978-0-444-64150-2.00009-5, ISBN 978-0-444-64150-2, PMID 32958196, https://linkinghub.elsevier.com/retrieve/pii/B9780444641502000095, retrieved 2023-03-26
- ↑ Galván, Adriana (2010-05-14). "Neural plasticity of development and learning". Human Brain Mapping 31 (6): 879–890. doi:10.1002/hbm.21029. PMID 20496379.
- ↑ "Developmental plasticity in respiratory control". Journal of Applied Physiology 94 (1): 375–389. January 2003. doi:10.1152/japplphysiol.00809.2002. PMID 12486025.
- ↑ "Spontaneous correlated activity in developing neural circuits". Neuron 22 (4): 653–656. April 1999. doi:10.1016/s0896-6273(00)80724-2. PMID 10230785.
- ↑ "Spontaneous network activity in the embryonic spinal cord regulates AMPAergic and GABAergic synaptic strength". Neuron 49 (4): 563–575. February 2006. doi:10.1016/j.neuron.2006.01.017. PMID 16476665.
- ↑ Arnold, Pieter A.; Kruuk, Loeske E. B.; Nicotra, Adrienne B. (2019-01-11). "How to analyse plant phenotypic plasticity in response to a changing climate". New Phytologist 222 (3): 1235–1241. doi:10.1111/nph.15656. ISSN 0028-646X. PMID 30632169.
- ↑ Nijhout, H. Frederik (March 1999). "Control Mechanisms of Polyphenic Development in Insects". BioScience 49 (3): 181–192. doi:10.2307/1313508.
- ↑ Yang, Chih-Hsiang; Andrew Pospisilik, John (2019-02-26). "Polyphenism – A Window Into Gene-Environment Interactions and Phenotypic Plasticity". Frontiers in Genetics 10: 132. doi:10.3389/fgene.2019.00132. ISSN 1664-8021. PMID 30863426.
- ↑ 39.0 39.1 Hales, Nicole R.; Schield, Drew R.; Andrew, Audra L.; Card, Daren C.; Walsh, Matthew R.; Castoe, Todd A. (2017). "Contrasting gene expression programs correspond with predator-induced phenotypic plasticity within and across generations in Daphnia". Molecular Ecology 26 (19): 5003–5015. doi:10.1111/mec.14213.
- ↑ 40.0 40.1 Zambre, Amod Mohan; Burns, Linnea; Suresh, Jayanti; Hegeman, Adrian D.; Snell-Rood, Emilie C. (2022). "Developmental plasticity in multimodal signals: light environment produces novel signalling phenotypes in a butterfly". Biology Letters 18 (8): 20220099. doi:10.1098/rsbl.2022.0099. ISSN 1744-957X. PMID 35975631.
- ↑ 41.0 41.1 Bock, Samantha L.; Smaga, Christopher R.; McCoy, Jessica A.; Parrott, Benjamin B. (2022). "Genome‐wide DNA methylation patterns harbour signatures of hatchling sex and past incubation temperature in a species with environmental sex determination". Molecular Ecology 31 (21): 5487–5505. doi:10.1111/mec.16670. ISSN 0962-1083. PMID 35997618.
Further reading
- "Temporal regulation of the expression locus of homeostatic plasticity". Journal of Neurophysiology 96 (4): 2127–2133. October 2006. doi:10.1152/jn.00107.2006. PMID 16760351.
- "Nicotine-induced plasticity during development: modulation of the cholinergic system and long-term consequences for circuits involved in attention and sensory processing". Neuropharmacology 56 (Suppl 1): 254–262. 2009. doi:10.1016/j.neuropharm.2008.07.020. PMID 18692078.
- "Chemical and Anatomical Plasticity of Brain". Science 146 (3644): 610–619. October 1964. doi:10.1126/science.146.3644.610. PMID 14191699. Bibcode: 1964Sci...146..610B.
- "How a child builds its brain: some lessons from animal studies of neural plasticity". Preventive Medicine 27 (2): 168–171. 1998. doi:10.1006/pmed.1998.0271. PMID 9578989.
- "Neuromodulation, development and synaptic plasticity". Canadian Journal of Experimental Psychology 53 (1): 45–61. March 1999. doi:10.1037/h0087299. PMID 10389489.
- "Developmental plasticity in respiratory control". Journal of Applied Physiology 94 (1): 375–389. January 2003. doi:10.1152/japplphysiol.00809.2002. PMID 12486025.
- "Activity-dependent structural plasticity". Brain Research Reviews 60 (2): 287–305. May 2009. doi:10.1016/j.brainresrev.2008.12.023. PMID 19162072.
- "Spontaneous correlated activity in developing neural circuits". Neuron 22 (4): 653–656. April 1999. doi:10.1016/s0896-6273(00)80724-2. PMID 10230785.
- "Spontaneous network activity in the embryonic spinal cord regulates AMPAergic and GABAergic synaptic strength". Neuron 49 (4): 563–575. February 2006. doi:10.1016/j.neuron.2006.01.017. PMID 16476665.
![]() | Original source: https://en.wikipedia.org/wiki/Developmental plasticity.
Read more |