Biology:Drosophila melanogaster
Drosophila melanogaster | |
---|---|
![]() | |
Fruit fly feeding off a banana | |
Scientific classification ![]() | |
Domain: | Eukaryota |
Kingdom: | Animalia |
Phylum: | Arthropoda |
Class: | Insecta |
Order: | Diptera |
Family: | Drosophilidae |
Genus: | Drosophila |
Subgenus: | Sophophora |
Species group: | melanogaster |
Species subgroup: | melanogaster |
Species complex: | melanogaster |
Species: | D. melanogaster
|
Binomial name | |
Drosophila melanogaster Meigen, 1830[1]
|
Drosophila melanogaster is a species of fly (an insect of the order Diptera) in the family Drosophilidae. The species is often referred to as the fruit fly or lesser fruit fly, or less commonly the "vinegar fly", "pomace fly",[lower-alpha 1][4] or "banana fly".[5] In the wild, D. melanogaster are attracted to rotting fruit and fermenting beverages, and are often found in orchards, kitchens and pubs.
Starting with Charles W. Woodworth's 1901 proposal of the use of this species as a model organism,[6][7] D. melanogaster continues to be widely used for biological research in genetics, physiology, microbial pathogenesis, and life history evolution. As of 2017, six Nobel Prizes have been awarded to drosophilists for their work using the insect.[8][9]
D. melanogaster is typically used in research owing to its rapid life cycle, relatively simple genetics with only four pairs of chromosomes, and large number of offspring per generation.[10] It was originally an African species, with all non-African lineages having a common origin.[11] Its geographic range includes all continents, including islands.[12] D. melanogaster is a common pest in homes, restaurants, and other places where food is served.[13]
Flies belonging to the family Tephritidae are also called "fruit flies". This can cause confusion, especially in the Mediterranean, Australia , and South Africa , where the Mediterranean fruit fly Ceratitis capitata is an economic pest.
Physical appearance
Wild type fruit flies are yellow-brown, with brick-red eyes and transverse black rings across the abdomen. The black portions of the abdomen are the inspiration for the species name (melanogaster = "black-bellied"). The brick-red color of the eyes of the wild type fly are due to two pigments:[14] xanthommatin, which is brown and is derived from tryptophan, and drosopterins, which are red and are derived from guanosine triphosphate.[14] They exhibit sexual dimorphism; females are about 2.5 mm (0.10 in) long; males are slightly smaller with darker backs. Males are easily distinguished from females based on colour differences, with a distinct black patch at the abdomen, less noticeable in recently emerged flies, and the sex combs (a row of dark bristles on the tarsus of the first leg). Furthermore, males have a cluster of spiky hairs (claspers) surrounding the reproducing parts used to attach to the female during mating. Extensive images are found at FlyBase.[15]
Drosophila melanogaster can be distinguished from related species by the following combination of features: gena ~1/10 diameter of eye at greatest vertical height; wing hyaline and with costal index 2.4; male protarsus with a single row of ~12 setae forming a sex comb; male epandrial posterior lobe small and nearly triangular; female abdominal tergite 6 with dark band running to its ventral margin; female oviscapt small, pale, without dorsodistal depression and with 12-13 peg-like outer ovisensilla.[16][17]
Drosophila melanogaster flies can sense air currents with the hairs on their backs. Their eyes are sensitive to slight differences in light intensity and will instinctively fly away when a shadow or other movement is detected.[18]
Lifecycle and reproduction
Under optimal growth conditions at 25 °C (77 °F), the D. melanogaster lifespan is about 50 days from egg to death.[19] The developmental period for D. melanogaster varies with temperature, as with many ectothermic species. The shortest development time (egg to adult), 7 days, is achieved at 28 °C (82 °F).[20][21] Development times increase at higher temperatures (11 days at 30 °C or 86 °F) due to heat stress. Under ideal conditions, the development time at 25 °C (77 °F) is 8.5 days,[20][21][22] at 18 °C (64 °F) it takes 19 days[20][21] and at 12 °C (54 °F) it takes over 50 days.[20][21] Under crowded conditions, development time increases,[23] while the emerging flies are smaller.[23][24] Females lay some 400 eggs (embryos), about five at a time, into rotting fruit or other suitable material such as decaying mushrooms and sap fluxes. Drosophila melanogaster is a holometabolous insect, so it undergoes a full metamorphosis. Their life cycle is broken down into 4 stages: embryo, larva, pupa, adult.[25] The eggs, which are about 0.5 mm long, hatch after 12–15 hours (at 25 °C or 77 °F).[20][21] The resulting larvae grow for about 4 days (at 25 °C) while molting twice (into second- and third-instar larvae), at about 24 and 48 h after hatching.[20][21] During this time, they feed on the microorganisms that decompose the fruit, as well as on the sugar of the fruit itself. The mother puts feces on the egg sacs to establish the same microbial composition in the larvae's guts that has worked positively for herself.[26] Then the larvae encapsulate in the puparium and undergo a 4-day-long metamorphosis (at 25 °C), after which the adults eclose (emerge).[20][21] File:Arrhythmia-Caused-by-a-Drosophila-Tropomyosin-Mutation-Is-Revealed-Using-a-Novel-Optical-Coherence-pone.0014348.s001.ogg
Males perform a sequence of five behavioral patterns to court females. First, males orient themselves while playing a courtship song by horizontally extending and vibrating their wings. Soon after, the male positions himself at the rear of the female's abdomen in a low posture to tap and lick the female genitalia. Finally, the male curls his abdomen and attempts copulation. Females can reject males by moving away, kicking, and extruding their ovipositor.[27] Copulation lasts around 15–20 minutes,[28] during which males transfer a few hundred, very long (1.76 mm) sperm cells in seminal fluid to the female.[29] Females store the sperm in a tubular receptacle and in two mushroom-shaped spermathecae; sperm from multiple matings compete for fertilization. A last male precedence is believed to exist; the last male to mate with a female sires about 80% of her offspring. This precedence was found to occur through both displacement and incapacitation.[30] The displacement is attributed to sperm handling by the female fly as multiple matings are conducted and is most significant during the first 1–2 days after copulation. Displacement from the seminal receptacle is more significant than displacement from the spermathecae.[30] Incapacitation of first male sperm by second male sperm becomes significant 2–7 days after copulation. The seminal fluid of the second male is believed to be responsible for this incapacitation mechanism (without removal of first male sperm) which takes effect before fertilization occurs.[30] The delay in effectiveness of the incapacitation mechanism is believed to be a protective mechanism that prevents a male fly from incapacitating his own sperm should he mate with the same female fly repetitively. Sensory neurons in the uterus of female D. melanogaster respond to a male protein, sex peptide, which is found in semen.[31] This protein makes the female reluctant to copulate for about 10 days after insemination. The signal pathway leading to this change in behavior has been determined. The signal is sent to a brain region that is a homolog of the hypothalamus and the hypothalamus then controls sexual behavior and desire.[31] Gonadotropic hormones in Drosophila maintain homeostasis and govern reproductive output via a cyclic interrelationship, not unlike the mammalian estrous cycle.[32] Sex peptide perturbs this homeostasis and dramatically shifts the endocrine state of the female by inciting juvenile hormone synthesis in the corpus allatum.[33]
D. melanogaster is often used for life extension studies, such as to identify genes purported to increase lifespan when mutated.[34] D. melanogaster is also used in studies of aging. Werner syndrome is a condition in humans characterized by accelerated aging. It is caused by mutations in the gene WRN that encodes a protein with essential roles in repair of DNA damage. Mutations in the D. melanogaster homolog of WRN also cause increased physiologic signs of aging, such as shorter lifespan, higher tumor incidence, muscle degeneration, reduced climbing ability, altered behavior and reduced locomotor activity.[35]
Females
Females become receptive to courting males about 8–12 hours after emergence.[36] Specific neuron groups in females have been found to affect copulation behavior and mate choice. One such group in the abdominal nerve cord allows the female fly to pause her body movements to copulate.[31] Activation of these neurons induces the female to cease movement and orient herself towards the male to allow for mounting. If the group is inactivated, the female remains in motion and does not copulate. Various chemical signals such as male pheromones often are able to activate the group.[31]
Also, females exhibit mate choice copying. When virgin females are shown other females copulating with a certain type of male, they tend to copulate more with this type of male afterwards than naïve females (which have not observed the copulation of others). This behavior is sensitive to environmental conditions, and females copulate less in bad weather conditions.[37]
Males
File:Turning-Males-On-Activation-of-Male-Courtship-Behavior-in-Drosophila-melanogaster-pone.0021144.s006.ogv D. melanogaster males exhibit a strong reproductive learning curve. That is, with sexual experience, these flies tend to modify their future mating behavior in multiple ways. These changes include increased selectivity for courting only intraspecifically, as well as decreased courtship times.
Sexually naïve D. melanogaster males are known to spend significant time courting interspecifically, such as with D. simulans flies. Naïve D. melanogaster will also attempt to court females that are not yet sexually mature, and other males. D. melanogaster males show little to no preference for D. melanogaster females over females of other species or even other male flies. However, after D. simulans or other flies incapable of copulation have rejected the males' advances, D. melanogaster males are much less likely to spend time courting nonspecifically in the future. This apparent learned behavior modification seems to be evolutionarily significant, as it allows the males to avoid investing energy into futile sexual encounters.[38]
In addition, males with previous sexual experience modify their courtship dance when attempting to mate with new females—the experienced males spend less time courting, so have lower mating latencies, meaning that they are able to reproduce more quickly. This decreased mating latency leads to a greater mating efficiency for experienced males over naïve males.[39] This modification also appears to have obvious evolutionary advantages, as increased mating efficiency is extremely important in the eyes of natural selection.
Polygamy
Both male and female D. melanogaster flies act polygamously (having multiple sexual partners at the same time).[40] In both males and females, polygamy results in a decrease in evening activity compared to virgin flies, more so in males than females.[40] Evening activity consists of those in which the flies participate other than mating and finding partners, such as finding food.[41] The reproductive success of males and females varies, because a female only needs to mate once to reach maximum fertility.[41] Mating with multiple partners provides no advantage over mating with one partner, so females exhibit no difference in evening activity between polygamous and monogamous individuals.[41] For males, however, mating with multiple partners increases their reproductive success by increasing the genetic diversity of their offspring.[41] This benefit of genetic diversity is an evolutionary advantage because it increases the chance that some of the offspring will have traits that increase their fitness in their environment.
The difference in evening activity between polygamous and monogamous male flies can be explained with courtship. For polygamous flies, their reproductive success increases by having offspring with multiple partners, and therefore they spend more time and energy on courting multiple females.[41] On the other hand, monogamous flies only court one female, and expend less energy doing so.[41] While it requires more energy for male flies to court multiple females, the overall reproductive benefits it produces has kept polygamy as the preferred sexual choice.[41]
The mechanism that affects courtship behavior in Drosophila is controlled by the oscillator neurons DN1s and LNDs.[42] Oscillation of the DN1 neurons was found to be effected by sociosexual interactions, and is connected to mating-related decrease of evening activity.[42]
Model organism in genetics
D. melanogaster remains one of the most studied organisms in biological research, particularly in genetics and developmental biology. It is also employed in studies of environmental mutagenesis.
History of use in genetic analysis
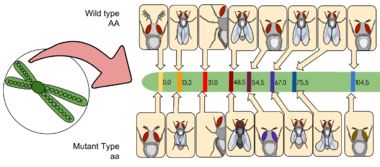
D. melanogaster was among the first organisms used for genetic analysis, and today it is one of the most widely used and genetically best-known of all eukaryotic organisms. All organisms use common genetic systems; therefore, comprehending processes such as transcription and replication in fruit flies helps in understanding these processes in other eukaryotes, including humans.[43]
Thomas Hunt Morgan began using fruit flies in experimental studies of heredity at Columbia University in 1910 in a laboratory known as the Fly Room. The Fly Room was cramped with eight desks, each occupied by students and their experiments. They started off experiments using milk bottles to rear the fruit flies and handheld lenses for observing their traits. The lenses were later replaced by microscopes, which enhanced their observations. Morgan and his students eventually elucidated many basic principles of heredity, including sex-linked inheritance, epistasis, multiple alleles, and gene mapping.[43]
D. melanogaster had historically been used in laboratories to study genetics and patterns of inheritance. However, D. melanogaster also has importance in environmental mutagenesis research, allowing researchers to study the effects of specific environmental mutagens.[44]
Reasons for use in laboratories
There are many reasons the fruit fly is a popular choice as a model organism:
- Its care and culture require little equipment, space, and expense even when using large cultures.
- It can be safely and readily anesthetized (usually with ether, carbon dioxide gas, by cooling, or with products such as FlyNap).
- Its morphology is easy to identify once anesthetized.
- It has a short generation time (about 10 days at room temperature), so several generations can be studied within a few weeks.
- It has a high fecundity (females lay up to 100 eggs per day, and perhaps 2000 in a lifetime).[10]
- Males and females are readily distinguished, and virgin females can be easily identified by their light-colored, translucent abdomen, facilitating genetic crossing.
- The mature larva has giant chromosomes in the salivary glands called polytene chromosomes, "puffs", which indicate regions of transcription, hence gene activity. The under-replication of rDNA occurs resulting in only 20% of DNA compared to the brain. Compare to the 47%, less rDNA in Sarcophaga barbata ovaries.
- It has only four pairs of chromosomes – three autosomes, and one pair of sex chromosomes.
- Males do not show meiotic recombination, facilitating genetic studies.
- Recessive lethal "balancer chromosomes" carrying visible genetic markers can be used to keep stocks of lethal alleles in a heterozygous state without recombination due to multiple inversions in the balancer.
- The development of this organism—from fertilized egg to mature adult—is well understood.
- Genetic transformation techniques have been available since 1987. One approach of inserting foreign genes into the Drosophila genome involves P elements. The transposable P elements, also known as transposons, are segments of bacterial DNA that are transferred into the fly genome. Transgenic flies have already contributed to many scientific advances, e.g., modeling such human diseases as Parkinson's, neoplasia, obesity, and diabetes.
- Its complete genome was sequenced and first published in 2000.[45]
- Sexual mosaics can be readily produced, providing an additional tool for studying the development and behavior of these flies.[46]
Genetic markers

Genetic markers are commonly used in Drosophila research, for example within balancer chromosomes or P-element inserts, and most phenotypes are easily identifiable either with the naked eye or under a microscope. In the list of a few common markers below, the allele symbol is followed by the name of the gene affected and a description of its phenotype. (Note: Recessive alleles are in lower case, while dominant alleles are capitalised.)
- Cy1: Curly; the wings curve away from the body, flight may be somewhat impaired
- e1: Ebony; black body and wings (heterozygotes are also visibly darker than wild type)
- Sb1: Stubble; bristles are shorter and thicker than wild type
- w1: White; eyes lack pigmentation and appear white
- bw: Brown; eye color determined by various pigments combined.
- y1: Yellow; body pigmentation and wings appear yellow, the fly analog of albinism
Classic genetic mutations
Drosophila genes are traditionally named after the phenotype they cause when mutated. For example, the absence of a particular gene in Drosophila will result in a mutant embryo that does not develop a heart. Scientists have thus called this gene tinman, named after the Oz character of the same name.[48] Likewise changes in the Shavenbaby gene cause the loss of dorsal cuticular hairs in Drosophila sechellia larvae.[49] This system of nomenclature results in a wider range of gene names than in other organisms.
- b: black- The black mutation was discovered in 1910 by Thomas Hunt Morgan.[50] The black mutation results in a darker colored body, wings, veins, and segments of the fruit fly's leg.[51] This occurs due to the fly's inability to create beta-alanine, a beta amino acid.[50] The phenotypic expression of this mutation varies based on the genotype of the individual; for example, whether the specimen is homozygotic or heterozygotic results in a darker or less dark appearance.[51] This genetic mutation is x-linked recessive.[52]
- bw: brown- The brown eye mutation results from inability to produce or synthesize pteridine (red) pigments, due to a point mutation on chromosome II.[53] When the mutation is homozygous, the pteridine pigments are unable to be synthesized because in the beginning of the pteridine pathway, a defective enzyme is being coded by homozygous recessive genes.[54][unreliable source?] In all, mutations in the pteridine pathway produces a darker eye color, hence the resulting color of the biochemical defect in the pteridine pathway being brown.
- m: miniature- One of the first records of the miniature mutation of wings was also made by Thomas Hunt Morgan in 1911. He described the wings as having a similar shape as the wild-type phenotype. However, their miniature designation refers to the lengths of their wings, which do not stretch beyond their body and, thus, are notably shorter than the wild-type length. He also noted its inheritance is connected to the sex of the fly and could be paired with the inheritance of other sex-determined traits such as white eyes.[55] The wings may also demonstrate other characteristics deviant from the wild-type wing, such as a duller and cloudier color.[56] Miniature wings are 1.5x shorter than wild-type but are believed to have the same number of cells. This is due to the lack of complete flattening by these cells, making the overall structure of the wing seem shorter in comparison. The pathway of wing expansion is regulated by a signal-receptor pathway, where the neurohormone bursicon interacts with its complementary G protein-coupled receptor; this receptor drives one of the G-protein subunits to signal further enzyme activity and results in development in the wing, such as apoptosis and growth.[57]
- se: sepia- The eye color of the sepia mutant is sepia, a reddish-brown color. In wild-type flies, ommochromes (brown) and drosopterins (red) give the eyes the typical red color.[58][59] The drosopterins are made via a pathway that involves a pyrimidodiazepine synthase,[60] which is encoded on chromosome 3L. The gene has a premature stop codon in sepia flies, so that the flies cannot produce the pyrimidodiazepine synthase and thus no red pigment, so that the eyes stay sepia.[58] The sepia allele is recessive and thus offspring from sepia flies and homozygous wild type flies, has red eyes. The sepia phenotype does not depend on the sex of the fly.[61]
- v: vermilion- The vermilion mutants cannot produce the brown ommochromes leaving the red drosopterins so that the eyes are vermilion colored (a radiant red) compared to a wild-type D. melanogaster. The vermilion mutation is sex-linked and recessive. The gene that is defect lies on the X chromosome.[62] The brown ommochromes are synthesised from kynurenine, which is made from tryptophane. Vermilion flies cannot convert tryptophane into kynurenine and thus cannot make ommochromes, either.[62] Vermilion mutants live longer than wild-type flies. This longer life span may be associated with the reduced amount of tryptophan converted to kynurenine in vermilion flies.[63]
- vg: vestigial- A spontaneous mutation, discovered in 1919 by Thomas Morgan and Calvin Bridges. Vestigial wings are those not fully developed and that have lost function. Since the discovery of the vestigial gene in Drosophila melanogaster, there have been many discoveries of the vestigial gene in other vertebrates and their functions within the vertebrates.[64] The vestigial gene is considered to be one of the most important genes for wing formation, but when it becomes over expressed the issue of ectopic wings begin to form.[65] The vestigial gene acts to regulate the expression of the wing imaginal discs in the embryo and acts with other genes to regulate the development of the wings. A mutated vestigial allele removes an essential sequence of the DNA required for correct development of the wings.[66]
- w: white- Drosophila melanogaster wild type typically expresses a brick red eye color. The white eye mutation in fruit flies is caused due to the absence of two pigments associated with red and brown eye colors; peridines (red) and ommochromes (brown).[59] In January 1910, Thomas Hunt Morgan first discovered the white gene and denoted it as w. The discovery of the white-eye mutation by Morgan brought about the beginnings of genetic experimentation and analysis of Drosophila melanogaster. Hunt eventually discovered that the gene followed a similar pattern of inheritance related to the meiotic segregation of the X chromosome. He discovered that the gene was located on the X chromosome with this information. This led to the discovery of sex-linked genes and also to the discovery of other mutations in Drosophila melanogaster.[67] The white-eye mutation leads to several disadvantages in flies, such as a reduced climbing ability, shortened life span, and lowered resistance to stress when compared to wild type flies.[68] Drosophila melanogaster has a series of mating behaviors that enable them to copulate within a given environment and therefore contribute to their fitness. After Morgan's discovery of the white-eye mutation being sex-linked, a study led by Sturtevant (1915) concluded that white-eyed males were less successful than wild-type males in terms of mating with females.[69] It was found that the greater the density in eye pigmentation, the greater the success in mating for the males of Drosophila melanogaster.[69]
- y: yellow- The yellow gene is a genetic mutation known as Dmel\y within the widely used data base called FlyBase. This mutation can be easily identified by the atypical yellow pigment observed in the cuticle of the adult flies and the mouth pieces of the larva.[70] The y mutation comprises the following phenotypic classes: the mutants that show a complete loss of pigmentation from the cuticle (y-type) and other mutants that show a mosaic pigment pattern with some regions of the cuticle (wild type, y2-type).[71] The role of the yellow gene is diverse and is responsible for changes in behaviour, sex-specific reproductive maturation and, epigenetic reprogramming.[72] The y gene is an ideal gene to study as it is visibly clear when an organism has this gene, making it easier to understand the passage of DNA to offspring.[72]
Genome
![]() D. melanogaster chromosomes to scale with megabase-pair references oriented as in the National Center for Biotechnology Information database, centimorgan distances are approximate and estimated from the locations of selected mapped loci. | |
NCBI genome ID | 47 |
---|---|
Ploidy | diploid |
Number of chromosomes | 8 |
Year of completion | 2015 |
The genome of D. melanogaster (sequenced in 2000, and curated at the FlyBase database[45]) contains four pairs of chromosomes – an X/Y pair, and three autosomes labeled 2, 3, and 4. The fourth chromosome is relatively very small and therefore often ignored, aside from its important eyeless gene. The D. melanogaster sequenced genome of 139.5 million base pairs has been annotated[73] and contains around 15,682 genes according to Ensemble release 73. More than 60% of the genome appears to be functional non-protein-coding DNA[74] involved in gene expression control. Determination of sex in Drosophila occurs by the X of X chromosomes to autosomes, not because of the presence of a Y chromosome as in human sex determination. Although the Y chromosome is entirely heterochromatic, it contains at least 16 genes, many of which are thought to have male-related functions.[75]
There are three transferrin orthologs, all of which are dramatically divergent from those known in chordate models.[76]
Similarity to humans
A March 2000 study by National Human Genome Research Institute comparing the fruit fly and human genome estimated that about 60% of genes are conserved between the two species.[77] About 75% of known human disease genes have a recognizable match in the genome of fruit flies,[78] and 50% of fly protein sequences have mammalian homologs [citation needed]. An online database called Homophila is available to search for human disease gene homologues in flies and vice versa.[79]
Drosophila is being used as a genetic model for several human diseases including the neurodegenerative disorders Parkinson's, Huntington's, spinocerebellar ataxia and Alzheimer's disease.[80] The fly is also being used to study mechanisms underlying aging and oxidative stress, immunity, diabetes, and cancer, as well as drug abuse.[81][82][83]
Development
The life cycle of this insect has four stages: fertilized egg, larva, pupa, and adult.[12]
Embryogenesis in Drosophila has been extensively studied, as its small size, short generation time, and large brood size makes it ideal for genetic studies. It is also unique among model organisms in that cleavage occurs in a syncytium.

During oogenesis, cytoplasmic bridges called "ring canals" connect the forming oocyte to nurse cells. Nutrients and developmental control molecules move from the nurse cells into the oocyte. In the figure to the left, the forming oocyte can be seen to be covered by follicular support cells.
After fertilization of the oocyte, the early embryo (or syncytial embryo) undergoes rapid DNA replication and 13 nuclear divisions until about 5000 to 6000 nuclei accumulate in the unseparated cytoplasm of the embryo. By the end of the eighth division, most nuclei have migrated to the surface, surrounding the yolk sac (leaving behind only a few nuclei, which will become the yolk nuclei). After the 10th division, the pole cells form at the posterior end of the embryo, segregating the germ line from the syncytium. Finally, after the 13th division, cell membranes slowly invaginate, dividing the syncytium into individual somatic cells. Once this process is completed, gastrulation starts.[84]
Nuclear division in the early Drosophila embryo happens so quickly, no proper checkpoints exist, so mistakes may be made in division of the DNA. To get around this problem, the nuclei that have made a mistake detach from their centrosomes and fall into the centre of the embryo (yolk sac), which will not form part of the fly.
The gene network (transcriptional and protein interactions) governing the early development of the fruit fly embryo is one of the best understood gene networks to date, especially the patterning along the anteroposterior (AP) and dorsoventral (DV) axes (See under morphogenesis).[84]
The embryo undergoes well-characterized morphogenetic movements during gastrulation and early development, including germ-band extension, formation of several furrows, ventral invagination of the mesoderm, and posterior and anterior invagination of endoderm (gut), as well as extensive body segmentation until finally hatching from the surrounding cuticle into a first-instar larva.
During larval development, tissues known as imaginal discs grow inside the larva. Imaginal discs develop to form most structures of the adult body, such as the head, legs, wings, thorax, and genitalia. Cells of the imaginal disks are set aside during embryogenesis and continue to grow and divide during the larval stages—unlike most other cells of the larva, which have differentiated to perform specialized functions and grow without further cell division. At metamorphosis, the larva forms a pupa, inside which the larval tissues are reabsorbed and the imaginal tissues undergo extensive morphogenetic movements to form adult structures.
Developmental plasticity
Biotic and abiotic factors experienced during development will affect developmental resource allocation leading to phenotypic variation, also referred to as developmental plasticity.[85][86] As in all insects,[86] environmental factors can influence several aspects of development in Drosophila melanogaster.[87][88] Fruit flies reared under a hypoxia treatment experience decreased thorax length, while hyperoxia produces smaller flight muscles, suggesting negative developmental effects of extreme oxygen levels.[89] Circadian rhythms are also subject to developmental plasticity. Light conditions during development affect daily activity patterns in Drosophila melanogaster, where flies raised under constant dark or light are less active as adults than those raised under a 12-hour light/dark cycle.[90]
Temperature is one of the most pervasive factors influencing arthropod development. In Drosophila melanogaster temperature-induced developmental plasticity can be beneficial and/or detrimental.[91][92] Most often lower developmental temperatures reduce growth rates which influence many other physiological factors.[93] For example, development at 25 °C increases walking speed, thermal performance breadth, and territorial success, while development at 18 °C increases body mass, wing size, all of which are tied to fitness.[88][91] Moreover, developing at certain low temperatures produces proportionally large wings which improve flight and reproductive performance at similarly low temperatures (See acclimation).[94]
While certain effects of developmental temperature, like body size, are irreversible in ectotherms, others can be reversible.[86][95] When Drosophila melanogaster develop at cold temperatures they will have greater cold tolerance, but if cold-reared flies are maintained at warmer temperatures their cold tolerance decreases and heat tolerance increases over time.[95][96] Because insects typically only mate in a specific range of temperatures, their cold/heat tolerance is an important trait in maximizing reproductive output.[97]
While the traits described above are expected to manifest similarly across sexes, developmental temperature can also produce sex-specific effects in D. melanogaster adults.
- Females- Ovariole number is significantly affected by developmental temperature in D. melanogaster.[98] Egg size is also affected by developmental temperature, and exacerbated when both parents develop at warm temperatures (See Maternal effect).[91] Under stressful temperatures, these structures will develop to smaller ultimate sizes and decrease a female's reproductive output.[98][91] Early fecundity (total eggs laid in first 10 days post-eclosion) is maximized when reared at 25 °C (versus 17 °C and 29 °C) regardless of adult temperature.[99] Across a wide range of developmental temperatures, females tend to have greater heat tolerance than males.[100]
- Males- Stressful developmental temperatures will cause sterility in D. melanogaster males; although the upper temperature limit can be increased by maintaining strains at high temperatures (See acclimation).[92] Male sterility can be reversible if adults are returned to an optimal temperature after developing at stressful temperatures.[101] Male flies are smaller and more successful at defending food/oviposition sites when reared at 25 °C versus 18 °C; thus smaller males will have increased mating success and reproductive output.[88]
Sex determination
Drosophila flies have both X and Y chromosomes, as well as autosomes. Unlike humans, the Y chromosome does not confer maleness; rather, it encodes genes necessary for making sperm. Sex is instead determined by the ratio of X chromosomes to autosomes.[102] Furthermore, each cell "decides" whether to be male or female independently of the rest of the organism, resulting in the occasional occurrence of gynandromorphs.
X Chromosomes | Autosomes | Ratio of X:A | Sex |
---|---|---|---|
XXXX | AAAA | 1 | Normal Female |
XXX | AAA | 1 | Normal Female |
XXY | AA | 1 | Normal Female |
XXYY | AA | 1 | Normal Female |
XX | AA | 1 | Normal Female |
XY | AA | 0.50 | Normal Male |
X | AA | 0.50 | Normal Male (sterile) |
XXX | AA | 1.50 | Metafemale |
XXXX | AAA | 1.33 | Metafemale |
XX | AAA | 0.66 | Intersex |
X | AAA | 0.33 | Metamale |
Three major genes are involved in determination of Drosophila sex. These are sex-lethal, sisterless, and deadpan. Deadpan is an autosomal gene which inhibits sex-lethal, while sisterless is carried on the X chromosome and inhibits the action of deadpan. An AAX cell has twice as much deadpan as sisterless, so sex-lethal will be inhibited, creating a male. However, an AAXX cell will produce enough sisterless to inhibit the action of deadpan, allowing the sex-lethal gene to be transcribed to create a female.
Later, control by deadpan and sisterless disappears and what becomes important is the form of the sex-lethal gene. A secondary promoter causes transcription in both males and females. Analysis of the cDNA has shown that different forms are expressed in males and females. Sex-lethal has been shown to affect the splicing of its own mRNA. In males, the third exon is included which encodes a stop codon, causing a truncated form to be produced. In the female version, the presence of sex-lethal causes this exon to be missed out; the other seven amino acids are produced as a full peptide chain, again giving a difference between males and females.[103]
Presence or absence of functional sex-lethal proteins now go on to affect the transcription of another protein known as doublesex. In the absence of sex-lethal, doublesex will have the fourth exon removed and be translated up to and including exon 6 (DSX-M[ale]), while in its presence the fourth exon which encodes a stop codon will produce a truncated version of the protein (DSX-F[emale]). DSX-F causes transcription of Yolk proteins 1 and 2 in somatic cells, which will be pumped into the oocyte on its production.
Immunity
The D. melanogaster immune system can be divided into two responses: humoral and cell-mediated. The former is a systemic response mediated in large part through the toll and Imd pathways, which are parallel systems for detecting microbes. Other pathways including the stress response pathways JAK-STAT and P38, nutritional signalling via FOXO, and JNK cell death signalling are all involved in key physiological responses to infection. D. melanogaster has an organ called the "fat body", which is analogous to the human liver. The fat body is the primary secretory organ and produces key immune molecules upon infection, such as serine proteases and antimicrobial peptides (AMPs). AMPs are secreted into the hemolymph and bind infectious bacteria and fungi, killing them by forming pores in their cell walls or inhibiting intracellular processes. The cellular immune response instead refers to the direct activity of blood cells (hemocytes) in Drosophila, which are analogous to mammalian monocytes/macrophages. Hemocytes also possess a significant role in mediating humoral immune responses such as the melanization reaction.[104]
The immune response to infection can involve up to 2,423 genes, or 13.7% of the genome. Although the fly's transcriptional response to microbial challenge is highly specific to individual pathogens, Drosophila differentially expresses a core group of 252 genes upon infection with most bacteria. This core group of genes is associated with gene ontology categories such as antimicrobial response, stress response, secretion, neuron-like, reproduction, and metabolism among others.[105][106] Drosophila also possesses several immune mechanisms to both shape the microbiota and prevent excessive immune responses upon detection of microbial stimuli. For instance, secreted PGRPs with amidase activity scavenge and degrade immunostimulatory DAP-type PGN in order to block Imd activation.[107]
Unlike mammals, Drosophila have innate immunity but lack an adaptive immune response. However, the core elements of this innate immune response are conserved between humans and fruit flies. As a result, the fruit fly offers a useful model of innate immunity for disentangling genetic interactions of signalling and effector function, as flies do not have to contend with interference of adaptive immune mechanisms that could confuse results. Various genetic tools, protocols, and assays make Drosophila a classical model for studying the innate immune system,[108] which has even included immune research on the international space station.[109]
JAK-STAT signalling
Multiple elements of the Drosophila JAK-STAT signalling pathway bear direct homology to human JAK-STAT pathway genes. JAK-STAT signalling is induced upon various organismal stresses such as heat stress, dehydration, or infection. JAK-STAT induction leads to the production of a number of stress response proteins including Thioester-containing proteins (TEPs),[110] Turandots,[111] and the putative antimicrobial peptide Listericin.[112] The mechanisms through which many of these proteins act is still under investigation. For instance, the TEPs appear to promote phagocytosis of Gram-positive bacteria and the induction of the toll pathway. As a consequence, flies lacking TEPs are susceptible to infection by toll pathway challenges.[110] File:Drosophila-Embryos-as-Model-Systems-for-Monitoring-Bacterial-Infection-in-Real-Time-ppat.1000518.s001.ogv
The cellular response to infection
Circulating hemocytes are key regulators of infection. This has been demonstrated both through genetic tools to generate flies lacking hemocytes, or through injecting microglass beads or lipid droplets that saturate hemocyte ability to phagocytose a secondary infection.[113][114] Flies treated like this fail to phagocytose bacteria upon infection, and are correspondingly susceptible to infection.[115] These hemocytes derive from two waves of hematopoiesis, one occurring in the early embryo and one occurring during development from larva to adult.[116] However Drosophila hemocytes do not renew over the adult lifespan, and so the fly has a finite number of hemocytes that decrease over the course of its lifespan.[117] Hemocytes are also involved in regulating cell-cycle events and apoptosis of aberrant tissue (e.g. cancerous cells) by producing Eiger, a tumor necrosis factor signalling molecule that promotes JNK signalling and ultimately cell death and apoptosis.[118]
Behavioral genetics and neuroscience
In 1971, Ron Konopka and Seymour Benzer published "Clock mutants of Drosophila melanogaster", a paper describing the first mutations that affected an animal's behavior. Wild-type flies show an activity rhythm with a frequency of about a day (24 hours). They found mutants with faster and slower rhythms, as well as broken rhythms—flies that move and rest in random spurts. Work over the following 30 years has shown that these mutations (and others like them) affect a group of genes and their products that form a biochemical or biological clock. This clock is found in a wide range of fly cells, but the clock-bearing cells that control activity are several dozen neurons in the fly's central brain.
Since then, Benzer and others have used behavioral screens to isolate genes involved in vision, olfaction, audition, learning/memory, courtship, pain, and other processes, such as longevity.
Following the pioneering work of Alfred Henry Sturtevant[119] and others, Benzer and colleagues[46] used sexual mosaics to develop a novel fate mapping technique. This technique made it possible to assign a particular characteristic to a specific anatomical location. For example, this technique showed that male courtship behavior is controlled by the brain.[46] Mosaic fate mapping also provided the first indication of the existence of pheromones in this species.[120] Males distinguish between conspecific males and females and direct persistent courtship preferentially toward females thanks to a female-specific sex pheromone which is mostly produced by the female's tergites.
The first learning and memory mutants (dunce, rutabaga, etc.) were isolated by William "Chip" Quinn while in Benzer's lab, and were eventually shown to encode components of an intracellular signaling pathway involving cyclic AMP, protein kinase A, and a transcription factor known as CREB. These molecules were shown to be also involved in synaptic plasticity in Aplysia and mammals.[121]
The Nobel Prize in Physiology or Medicine for 2017 was awarded to Jeffrey C. Hall, Michael Rosbash, Michael W. Young for their works using fruit flies in understanding the "molecular mechanisms controlling the circadian rhythm".[122]
Male flies sing to the females during courtship using their wings to generate sound, and some of the genetics of sexual behavior have been characterized. In particular, the fruitless gene has several different splice forms, and male flies expressing female splice forms have female-like behavior and vice versa. The TRP channels nompC, nanchung, and inactive are expressed in sound-sensitive Johnston's organ neurons and participate in the transduction of sound.[123][124] Mutating the Genderblind gene, also known as CG6070, alters the sexual behavior of Drosophila, turning the flies bisexual.[125]
Flies use a modified version of Bloom filters to detect novelty of odors, with additional features including similarity of novel odor to that of previously experienced examples, and time elapsed since previous experience of the same odor.[126]
Aggression
As with most insects, aggressive behaviors between male flies commonly occur in the presence of courting a female and when competing for resources. Such behaviors often involve raising wings and legs towards the opponent and attacking with the whole body.[127] Thus, it often causes wing damage, which reduces their fitness by removing their ability to fly and mate.[128]
Acoustic communication
In order for aggression to occur, male flies produce sounds to communicate their intent. A 2017 study found that songs promoting aggression contain pulses occurring at longer intervals.[129] RNA sequencing from fly mutants displaying over-aggressive behaviors found more than 50 auditory-related genes (important for transient receptor potentials, Ca2+ signaling, and mechanoreceptor potentials) to be upregulated in the AB neurons located in Johnston's organ.[129] In addition, aggression levels were reduced when these genes were knocked out via RNA interference.[129] This signifies the major role of hearing as a sensory modality in communicating aggression.
Pheromone signaling
Other than hearing, another sensory modality that regulates aggression is pheromone signaling, which operates through either the olfactory system or the gustatory system depending on the pheromone.[130] An example is cVA, an anti-aphrodisiac pheromone used by males to mark females after copulation and to deter other males from mating.[131] This male-specific pheromone causes an increase in male-male aggression when detected by another male's gustatory system.[130] However, upon inserting a mutation that makes the flies irresponsive to cVA, no aggressive behaviors were seen.[132] This shows how there are multiple modalities for promoting aggression in flies.
Competition for food
Specifically, when competing for food, aggression occurs based on amount of food available and is independent of any social interactions between males.[133] Specifically, sucrose was found to stimulate gustatory receptor neurons, which was necessary to stimulate aggression.[133] However, once the amount of food becomes greater than a certain amount, the competition between males lowers.[133] This is possibly due to an over-abundance of food resources. On a larger scale, food was found to determine the boundaries of a territory since flies were observed to be more aggressive at the food's physical perimeter.
Effect of sleep deprivation
However, like most behaviors requiring arousal and wakefulness, aggression was found to be impaired via sleep deprivation. Specifically, this occurs through the impairment of Octopamine and dopamine signaling, which are important pathways for regulating arousal in insects.[134][135] Due to reduced aggression, sleep-deprived male flies were found to be disadvantaged at mating compared to normal flies.[135] However, when octopamine agonists were administered upon these sleep-deprived flies, aggression levels were seen to be increased and sexual fitness was subsequently restored.[135] Therefore, this finding implicates the importance of sleep in aggression between male flies.
Vision
The compound eye of the fruit fly contains 760 unit eyes or ommatidia, and are one of the most advanced among insects. Each ommatidium contains eight photoreceptor cells (R1-8), support cells, pigment cells, and a cornea. Wild-type flies have reddish pigment cells, which serve to absorb excess blue light so the fly is not blinded by ambient light. Eye color genes regulate cellular vesicular transport. The enzymes needed for pigment synthesis are then transported to the cell's pigment granule, which holds pigment precursor molecules.[59]
Each photoreceptor cell consists of two main sections, the cell body and the rhabdomere. The cell body contains the nucleus, while the 100-μm-long rhabdomere is made up of toothbrush-like stacks of membrane called microvilli. Each microvillus is 1–2 μm in length and about 60 nm in diameter.[136] The membrane of the rhabdomere is packed with about 100 million opsin molecules, the visual protein that absorbs light. The other visual proteins are also tightly packed into the microvilli, leaving little room for cytoplasm.
Opsins and spectral sensitivity

The genome of Drosophila encodes seven opsins,[138] five of those are expressed in the omatidia of the eye. The photoreceptor cells R1-R6 express the opsin Rh1,[139] which absorbs maximally blue light (around 480 nm),[140][141][142] however the R1-R6 cells cover a broader range of the spectrum than an opsin would allow due to a sensitising pigment[143][144] that adds two sensitivity maxima in the UV-range (355 and 370 nm).[142] The R7 cells come in two types with yellow and pale rhabdomeres (R7y and R7p).[145][146] The pale R7p cells express the opsin Rh3,[147][148] which maximally absorbs UV-light (345 nm).[149] The R7p cells are strictly paired with the R8p cells that express Rh5,[148] which maximally absorbs violet light (437 nm).[142] The other, the yellow R7y cells express a blue-absorbing screening pigment[145] and the opsin Rh4,[150] which maximally absorbs UV-light (375 nm).[149] The R7y cells are strictly paired with R8y cells that express Rh6,[151] which maximally absorbs UV-light (508 nm).[142] In a subset of omatidia both R7 and R8 cells express the opsin Rh3.[148]
However, these absorption maxima of the opsins where measured in white eyed flies without screening pigments (Rh3-Rh6),[149][142] or from the isolated opsin directly (Rh1).[140] Those pigments reduce the light that reaches the opsins depending on the wavelength. Thus in fully pigmented flies, the effective absorption maxima of opsins differs and thus also the sensitivity of their photoreceptor cells. With screening pigment, the opsin Rh3 is short wave shifted from 345 nm[lower-alpha 2] to 330 nm and Rh4 from 375 nm to 355 nm. Whether screening pigment is present does not make a practical difference for the opsin Rh5 (435 nm and 437 nm), while the opsin R6 is long wave shifted by 92 nm from 508 nm to 600 nm.[137]
Additionally of the opsins of the eye, Drosophila has two more opsins: The ocelli express the opsin Rh2,[152][153] which maximally absorbs violet light (~420 nm).[153] And the opsin Rh7, which maximally absorbs UV-light (350 nm) with an unusually long wavelength tail up to 500 nm. The long tail disappears if a lysine at position 90 is replaced by glutamic acid. This mutant then absorbs maximally violet light (450 nm).[154] The opsin Rh7 entrains with cryptochrome the circadian rhythm of Drosophila to the day-night-cycle in the central pacemaker neurons.[155]
Each Drosophila opsin binds the carotenoid chromophore 11-cis-3-hydroxyretinal via a lysine.[156][157] This lysine is conserved in almost all opsins, only a few opsins have lost it during evolution.[158] Opsins without it are not light sensitive.[159][160][161] In particular, the Drosophila opsins Rh1, Rh4, and Rh7 function not only as photoreceptors, but also as chemoreceptors for aristolochic acid. These opsins still have the lysine like other opsins. However, if it is replaced by an arginine in Rh1, then Rh1 loses light sensitivity but still responds to aristolochic acid. Thus, the lysine is not needed for Rh1 to function as chemoreceptor.[160]
Spectral sensitivities of Drosophila melanogaster opsins in white eyed flies. The sensitivities of Rh3–R6 are modelled with opsin templates and sensitivity estimates from Salcedo et al. (1999).[142] The opsin Rh1 (redrawn from Salcedo et al.[142]) has a characteristic shape as it is coupled to a UV-sensitising pigment.
Phototransduction
As in vertebrate vision, visual transduction in invertebrates occurs via a G protein-coupled pathway. However, in vertebrates, the G protein is transducin, while the G protein in invertebrates is Gq (dgq in Drosophila). When rhodopsin (Rh) absorbs a photon of light its chromophore, 11-cis-3-hydroxyretinal, is isomerized to all-trans-3-hydroxyretinal. Rh undergoes a conformational change into its active form, metarhodopsin. Metarhodopsin activates Gq, which in turn activates a phospholipase Cβ (PLCβ) known as NorpA.[162]
PLCβ hydrolyzes phosphatidylinositol (4,5)-bisphosphate (PIP2), a phospholipid found in the cell membrane, into soluble inositol triphosphate (IP3) and diacylglycerol (DAG), which stays in the cell membrane. DAG, a derivative of DAG, or PIP2 depletion cause a calcium-selective ion channel known as transient receptor potential (TRP) to open and calcium and sodium flows into the cell.[163] IP3 is thought to bind to IP3 receptors in the subrhabdomeric cisternae, an extension of the endoplasmic reticulum, and cause release of calcium, but this process does not seem to be essential for normal vision.[162]
Calcium binds to proteins such as calmodulin (CaM) and an eye-specific protein kinase C (PKC) known as InaC. These proteins interact with other proteins and have been shown to be necessary for shut off of the light response. In addition, proteins called arrestins bind metarhodopsin and prevent it from activating more Gq. A sodium-calcium exchanger known as CalX pumps the calcium out of the cell. It uses the inward sodium gradient to export calcium at a stoichiometry of 3 Na+/ 1 Ca++.[164]
TRP, InaC, and PLC form a signaling complex by binding a scaffolding protein called InaD. InaD contains five binding domains called PDZ domain proteins, which specifically bind the C termini of target proteins. Disruption of the complex by mutations in either the PDZ domains or the target proteins reduces the efficiency of signaling. For example, disruption of the interaction between InaC, the protein kinase C, and InaD results in a delay in inactivation of the light response.
Unlike vertebrate metarhodopsin, invertebrate metarhodopsin can be converted back into rhodopsin by absorbing a photon of orange light (580 nm).
About two-thirds of the Drosophila brain is dedicated to visual processing.[165] Although the spatial resolution of their vision is significantly worse than that of humans, their temporal resolution is around 10 times better.
Grooming
Drosophila are known to exhibit grooming behaviors that are executed in a predictable manner. Drosophila consistently begin a grooming sequence by using their front legs to clean the eyes, then the head and antennae. Using their hind legs, Drosophila proceed to groom their abdomen, and finally the wings and thorax. Throughout this sequence, Drosophila periodically rub their legs together to get rid of excess dust and debris that accumulates during the grooming process.[166]
Grooming behaviors have been shown to be executed in a suppression hierarchy. This means that grooming behaviors that occur at the beginning of the sequence prevent those that come later in the sequence from occurring simultaneously, as the grooming sequence consists of mutually exclusive behaviors.[167][168] This hierarchy does not prevent Drosophila from returning to grooming behaviors that have already been accessed in the grooming sequence.[167] The order of grooming behaviors in the suppression hierarchy is thought to be related to the priority of cleaning a specific body part. For example, the eyes and antennae are likely executed early on in the grooming sequence to prevent debris from interfering with the function of D. melanogaster's sensory organs.[167][168]
Walking

Like many other hexapod insects, Drosophila typically walk using a tripod gait.[170] This means that three of the legs swing together while the other three remain stationary, or in stance. Specifically, the middle leg moves in phase with the contralateral front and hind legs. However, variability around the tripod configuration exists along a continuum, meaning that flies do not exhibit distinct transitions between different gaits.[171] At fast walking speeds, the walking configuration is mostly tripod (3 legs in stance), but at slower walking speeds, flies are more likely to have four (tetrapod) or five legs in stance (wave).[172][173] These transitions may help to optimize static stability.[174] Because flies are so small, inertial forces are negligible compared with the elastic forces of their muscles and joints or the viscous forces of the surrounding air.[175]
Flight
Flies fly via straight sequences of movement interspersed by rapid turns called saccades.[176] During these turns, a fly is able to rotate 90° in less than 50 milliseconds.[176]
Characteristics of Drosophila flight may be dominated by the viscosity of the air, rather than the inertia of the fly body, but the opposite case with inertia as the dominant force may occur.[176] However, subsequent work showed that while the viscous effects on the insect body during flight may be negligible, the aerodynamic forces on the wings themselves actually cause fruit flies' turns to be damped viscously.[177]
Connectome
Drosophila is one of the few animals (C. elegans being another) where detailed neural circuits (a connectome) are available.
A high-level connectome, at the level of brain compartments and interconnecting tracts of neurons, exists for the full fly brain.[178] A version of this is available online.[179]
Detailed circuit-level connectomes exist for the lamina[180][181] and a medulla[182] column, both in the visual system of the fruit fly, and the alpha lobe of the mushroom body.[183]
In May 2017 a paper published in bioRxiv presented an electron microscopy image stack of the whole adult female brain at synaptic resolution. The volume is available for sparse tracing of selected circuits.[184][185] Since then, multiple datasets have been collected including a dense connectome of half the central brain of Drosophila in 2020,[186][187] and a dense connectome of the entire female adult nerve cord in 2021.[188] Generally, these datasets are acquired by sectioning the tissue (e.g. the brain) into thin sections (on order of ten or hundreds of nanometers). Each section is then imaged using an electron microscope and these images are stitched and aligned together to create a 3D image volume. The methods used in reconstruction and initial analysis of the such datasets followed.[189] Due to advancements in deep learning, automated methods for image segmentation have made large scale reconstruction providing dense reconstructions of all the neurites within the volume.[190] Furthermore, the resolution of electron microscopy illuminates ultrastructural variations between neurons as well as the location of individual synapses, thereby providing a wiring diagram of synaptic connectivity between all neurites within the given dataset.
In 2023, the complete map of a Drosophila larval brain at the synapse level, and an analysis of its architecture was published. The larval brain consists of 3016 neurons and 548,000 synaptic sites,[191] whereas the adult brain has about 150,000 neurons and 150 million synapses.
Misconceptions
Drosophila is sometimes referred to as a pest due to its tendency to live in human settlements where fermenting fruit is found. Flies may collect in homes, restaurants, stores, and other locations.[13] The name and behavior of this species of fly have led to the misconception that it is a biological security risk in Australia and elsewhere. While other "fruit fly" species do pose a risk, D. melanogaster is attracted to fruit that is already rotting, rather than causing fruit to rot.[192][193]
See also
- Animal testing on invertebrates
- Eating behavior in Insects (Measurement)
- Fruit flies in space
- Genetically modified insect
- Gynandromorphism
- JETLAG gene
- List of Drosophila databases
- Spätzle (gene)
- Transgenesis
- Zebrafish – another widely used model organism in scientific research
Notes
- ↑ "Vinegar fly" is preferred by a handful of recent publications as being a more accurate description than "fruit fly".[2][3][4]
- ↑ Sharkey et al.[137] give the absorption maximum of Rh3 as 334 nm in their result section. However, in the introduction and the material and methods section they give it as 345 nm. For both values, they cite Feiler et al., who reported 345 nm only.[149] Therefore, this seems to be a mistake and they probably meant there 345 nm, too.
References
- ↑ (in de) Systematische Beschreibung der bekannten europäischen zweiflügeligen Insekten. (Volume 6). Schulz-Wundermann. 1830. https://dlib.stanford.edu:6521/text1/dd-ill/insekten6.pdf.
- ↑ "Drosophila | insect genus" (in en). https://www.britannica.com/animal/Drosophila.
- ↑ "Vinegar Flies" (in en). https://extension.psu.edu/vinegar-flies.
- ↑ 4.0 4.1 "It really is not a fruit fly". Genetics 162 (1): 1–3. September 2002. doi:10.1093/genetics/162.1.1. PMID 12242218.
- ↑ Schilthuizen, Menno (April 28, 2015). "Semen's Chemical Cocktail Can Hijack a Mate's Brain". https://www.discovermagazine.com/health/semens-chemical-cocktail-can-hijack-a-mates-brain. "Even in the ejaculate of the lowly banana fly Drosophila melanogaster, researchers have identified no fewer than 133 different kinds of proteins."
- ↑ T.H. Morgan's Nobel Prize biography mentioning C. W. Woodworth
- ↑ (in en) Charles W. Woodworth: The Remarkable Life of U.C.'s First Entomologist (1st ed.). Brian Holden Publishing. 2015-01-01. pp. 135–137. ISBN 978-0-9864105-3-6.
- ↑ "Nobel Prizes". 7 October 2017. https://www.theguardian.com/science/2017/oct/07/fruit-fly-fascination-nobel-prizes-genetics.
- ↑ "FruitFly-ResearchGate". https://www.researchgate.net/publication/321176879.
- ↑ 10.0 10.1 "Drosophila melanogaster: The Fruit Fly". Encyclopedia of genetics. USA: Fitzroy Dearborn Publishers, I. 2001-06-23. p. 157. ISBN 978-1-884964-34-3. https://books.google.com/books?id=JjLWYKqehRsC&q=drosophila+eggs+day+lifetime&pg=PA157. Retrieved 2009-07-01.
- ↑ "Non-African populations of Drosophila melanogaster have a unique origin". Molecular Biology and Evolution 21 (8): 1482–91. August 2004. doi:10.1093/molbev/msh089. PMID 15014160.
- ↑ 12.0 12.1 "The secret lives of Drosophila flies" (in en). eLife 4. June 2015. doi:10.7554/eLife.06793. PMID 26041333.
- ↑ 13.0 13.1 "Vinegar Flies, Drosophila species, Family: Drosophilidae". Department of Entomology, College of Agricultural Sciences, Pennsylvania State University. 2017. http://ento.psu.edu/extension/factsheets/vinegar-flies.
- ↑ 14.0 14.1 "ABC transporters involved in transport of eye pigment precursors in Drosophila melanogaster". ABC Transporters: Biochemical, Cellular, and Molecular Aspects. Methods in Enzymology. 292. Academic Press. 1998-01-01. pp. 213–24. doi:10.1016/S0076-6879(98)92017-1. ISBN 978-0-12-182193-7.
- ↑ "FlyBase: A database of Drosophila genes and genomes". Genetics Society of America. 2009. http://flybase.bio.indiana.edu/.
- ↑ Yuzuki, Keven; Tidon, Rosana (2020). "Identification key for drosophilid species (Diptera, Drosophilidae) exotic to the Neotropical Region and occurring in Brazil". Revista Brasileira de Entomologia 64 (1). doi:10.1590/1806-9665-rbent-2019-100. ISSN 1806-9665. http://www.scielo.br/scielo.php?script=sci_arttext&pid=S0085-56262020000100205&tlng=en.
- ↑ Miller, M. E.; Marshall, S. A.; Grimaldi, D. A. (2017). "A Review of the Species of Drosophila (Diptera: Drosophilidae) and Genera of Drosophilidae of Northeastern North America". Canadian Journal of Arthropod Identification 31. doi:10.3752/cjai.2017.31. https://cjai.biologicalsurvey.ca/articles/mmg-31/.
- ↑ "Drosophila Melanogaster". Animal Diversity Web. 2000. https://animaldiversity.org/accounts/Drosophila_melanogaster/.
- ↑ "Measurement of lifespan in Drosophila melanogaster". Journal of Visualized Experiments (71). January 2013. doi:10.3791/50068. PMID 23328955.
- ↑ 20.0 20.1 20.2 20.3 20.4 20.5 20.6 Ashburner M, Wright TRF, ed (1978). "The laboratory culture of Drosophila". The genetics and biology of Drosophila. 2A. Academic Press. pp. 1–81.
- ↑ 21.0 21.1 21.2 21.3 21.4 21.5 21.6 Drosophila: A Laboratory Handbook. (2nd ed.). Cold Spring Harbor Laboratory Press. 2005. pp. 162–4. ISBN 978-0-87969-706-8.
- ↑ Bloomington Drosophila Stock Center at Indiana University: Basic Methods of Culturing Drosophila
- ↑ 23.0 23.1 "An analytical study of population growth in Drosophila melanogaster". Ecological Monographs 20 (3): 173–206. 1950. doi:10.2307/1948580. Bibcode: 1950EcoM...20..173C.
- ↑ "An analysis of factors which determine success in competition for food among larvae of Drosophila melanogaster". Archives Néerlandaises de Zoologie 14 (2): 200–281. 1961. doi:10.1163/036551661X00061.
- ↑ "Drosophila melanogaster as a Model System to Study Mitochondrial Biology". Mitochondria. Methods in Molecular Biology. 372. 2007. pp. 33–49. doi:10.1007/978-1-59745-365-3_3. ISBN 978-1-58829-667-2.
- ↑ "Frequent replenishment sustains the beneficial microbiome of Drosophila melanogaster". mBio 4 (6): e00860-13. November 2013. doi:10.1128/mBio.00860-13. PMID 24194543.
- ↑ "Rejection Responses by Female Drosophila melanogaster: Their Ontogeny, Causality and Effects upon the Behaviour of the Courting Male". Behaviour 44 (1/2): 142–166. 1973. doi:10.1163/156853973x00364.
- ↑ "Effect of laboratory acclimation on the variation of reproduction-related characters in Drosophila melanogaster". The Journal of Experimental Biology 213 (Pt 13): 2322–31. July 2010. doi:10.1242/jeb.041566. PMID 20543131.
- ↑ "9: Fertilization in Drosophila". Developmental Biology (8th ed.). Sinauer Associates. 2006. ISBN 978-0-87893-250-4. http://8e.devbio.com/article.php?ch=9&id=87.
- ↑ 30.0 30.1 30.2 "Sperm competition between Drosophila males involves both displacement and incapacitation". Nature 400 (6743): 449–52. July 1999. doi:10.1038/22755. PMID 10440373. Bibcode: 1999Natur.400..449P.
- ↑ 31.0 31.1 31.2 31.3 "Fruit fly research may reveal what happens in female brains during courtship, mating". https://www.sciencedaily.com/releases/2014/07/140702122424.htm.
- ↑ "Drosophila melanogaster". Proceedings of the National Academy of Sciences of the United States of America 114 (19): E3849–E3858. May 2017. doi:10.1073/pnas.1620760114. PMID 28439025.
- ↑ "Sex-peptide activates juvenile hormone biosynthesis in the Drosophila melanogaster corpus allatum". Archives of Insect Biochemistry and Physiology 32 (3–4): 363–74. 1996. doi:10.1002/(SICI)1520-6327(1996)32:3/4<363::AID-ARCH9>3.0.CO;2-T. PMID 8756302.
- ↑ "The Genomic Basis of Postponed Senescence in Drosophila melanogaster". PLOS ONE 10 (9): e0138569. 2015. doi:10.1371/journal.pone.0138569. PMID 26378456. Bibcode: 2015PLoSO..1038569C.
- ↑ "Evidence for premature aging in a Drosophila model of Werner syndrome". Experimental Gerontology 127: 110733. November 2019. doi:10.1016/j.exger.2019.110733. PMID 31518666.
- ↑ "Investment in testes and the cost of making long sperm in Drosophila". American Naturalist 148: 57–80. 1996. doi:10.1086/285911.
- ↑ "Drosophila mate copying correlates with atmospheric pressure in a speed learning situation.". Animal Behaviour 121: 163–174. 2016. doi:10.1016/j.anbehav.2016.08.022.
- ↑ "Male fruit flies learn to avoid interspecific courtship". Behavioral Ecology 15 (4): 695–698. 2004. doi:10.1093/beheco/arh068.
- ↑ "Sexual experience enhances Drosophila melanogaster male mating behavior and success". PLOS ONE 9 (5): e96639. 2014. doi:10.1371/journal.pone.0096639. PMID 24805129. Bibcode: 2014PLoSO...996639S.
- ↑ 40.0 40.1 "Successive Polygamy". Behaviour 3 (1): 256–273. 1951. doi:10.1163/156853951x00296.
- ↑ 41.0 41.1 41.2 41.3 41.4 41.5 41.6 "Effects of polygamy on the activity/rest rhythm of male fruit flies Drosophila melanogaster". Die Naturwissenschaften 102 (1–2): 1252. February 2015. doi:10.1007/s00114-014-1252-5. PMID 25604736. Bibcode: 2015SciNa.102....3V.
- ↑ 42.0 42.1 "Intra-sexual selection in Drosophila". Heredity 2 (Pt. 3): 349–68. December 1948. doi:10.1038/hdy.1948.21. PMID 18103134.
- ↑ 43.0 43.1 Genetics: A Conceptual Approach (2nd ed.). W. H. Freeman. 2004. ISBN 978-0-7167-8881-2. https://archive.org/details/geneticsconceptu0000unse.
- ↑ "The use of Drosophila melanogaster in tests for environmental mutagens". Mutation Research 85 (3): 141–6. June 1981. doi:10.1016/0165-1161(81)90029-7. PMID 6790982.
- ↑ 45.0 45.1 "The genome sequence of Drosophila melanogaster". Science 287 (5461): 2185–95. March 2000. doi:10.1126/science.287.5461.2185. PMID 10731132. Bibcode: 2000Sci...287.2185..
- ↑ 46.0 46.1 46.2 "Mapping of behaviour in Drosophila mosaics". Nature 240 (5383): 527–35. December 1972. doi:10.1038/240527a0. PMID 4568399. Bibcode: 1972Natur.240..527H.
- ↑ Exploiting emerging DNA sequencing technologies to study genomic rearrangements. 2018. doi:10.11588/heidok.00024506. https://archiv.ub.uni-heidelberg.de/volltextserver/24506/. Retrieved 2021-06-28.
- ↑ "tinman and bagpipe: two homeo box genes that determine cell fates in the dorsal mesoderm of Drosophila". Genes & Development 7 (7B): 1325–40. July 1993. doi:10.1101/gad.7.7b.1325. PMID 8101173.
- ↑ "The structure and evolution of cis-regulatory regions: the shavenbaby story". Philosophical Transactions of the Royal Society of London. Series B, Biological Sciences 368 (1632): 20130028. December 2013. doi:10.1098/rstb.2013.0028. PMID 24218640.
- ↑ 50.0 50.1 "The Drosophila black enigma: the molecular and behavioural characterization of the black1 mutant allele". Gene 351: 131–42. May 2005. doi:10.1016/j.gene.2005.03.013. PMID 15878647. https://epub.uni-regensburg.de/28573/1/brembs.pdf.
- ↑ 51.0 51.1 "FlyBase Gene Report: Dmel\b". http://flybase.org/reports/FBgn0000153#redbook_container.
- ↑ "Intergenic suppression of the black mutation of Drosophila melanogaster". Molecular & General Genetics 183 (1): 102–6. September 1981. doi:10.1007/bf00270146. PMID 6799739.
- ↑ "The development of pigment granules in the eyes of wild type and mutant Drosophila melanogaster". The Journal of Cell Biology 29 (2): 223–49. May 1966. doi:10.1083/jcb.29.2.223. PMID 5961338.
- ↑ "TEACHER REFERENCE PAGES-FLY EYE PIGMENTS LAB". https://www.oxy.edu/sites/default/files/assets/TOPS/FLYEYE-T.pdf.
- ↑ "The Origin of Nine Wing Mutations in Drosophila". Science 33 (848): 496–9. March 1911. doi:10.1126/science.33.848.496. PMID 17774436. Bibcode: 1911Sci....33..496M.
- ↑ "FlyBase Gene Report: Dmel\m". http://flybase.org/reports/FBgn0002577.html.
- ↑ "The downregulation of the miniature gene does not replicate miniature loss-of-function phenotypes in Drosophila melanogaster wing to the full extent". TSitologiia I Genetika 47 (2): 77–81. Mar–Apr 2013. PMID 23745366.
- ↑ 58.0 58.1 "Identification and characteristics of the structural gene for the Drosophila eye colour mutant sepia, encoding PDA synthase, a member of the omega class glutathione S-transferases". The Biochemical Journal 398 (3): 451–60. September 2006. doi:10.1042/BJ20060424. PMID 16712527.
- ↑ 59.0 59.1 59.2 "An Eye on Trafficking Genes: Identification of Four Eye Color Mutations in Drosophila". G3 6 (10): 3185–3196. October 2016. doi:10.1534/g3.116.032508. PMID 27558665.
- ↑ "Purification and properties of the enzymes from Drosophila melanogaster that catalyze the conversion of dihydroneopterin triphosphate to the pyrimidodiazepine precursor of the drosopterins". J. Biol. Chem. 259 (22): 14121–7. 1984. doi:10.1016/S0021-9258(18)89865-9. PMID 6438092.
- ↑ "Inheritance Patterns in Drosophila Melanogaster". https://www.ukessays.com/essays/biology/investigating-patterns-of-inheritence.php.
- ↑ 62.0 62.1 "Mutant Isoalleles at the Vermilion Locus in Drosophila Melanogaster". Proceedings of the National Academy of Sciences of the United States of America 38 (4): 300–5. April 1952. doi:10.1073/pnas.38.4.300. PMID 16589094. Bibcode: 1952PNAS...38..300G.
- ↑ "The extended life span of Drosophila melanogaster eye-color (white and vermilion) mutants with impaired formation of kynurenine". Journal of Neural Transmission 117 (1): 23–26. January 2010. doi:10.1007/s00702-009-0341-7. PMID 19941150.
- ↑ "From vestigial to vestigial-like: the Drosophila gene that has taken wing". Development Genes and Evolution 226 (4): 297–315. July 2016. doi:10.1007/s00427-016-0546-3. PMID 27116603.
- ↑ "What serial homologs can tell us about the origin of insect wings". F1000Research 6: 268. 2017-03-14. doi:10.12688/f1000research.10285.1. PMID 28357056.
- ↑ "Control of Drosophila wing and haltere development by the nuclear vestigial gene product". Genes & Development 5 (12B): 2481–95. December 1991. doi:10.1101/gad.5.12b.2481. PMID 1752439.
- ↑ "2010: A century of Drosophila genetics through the prism of the white gene". Genetics 184 (1): 3–7. January 2010. doi:10.1534/genetics.109.110015. PMID 20061564.
- ↑ "rosophila melanogaster White Mutant w1118 Undergo Retinal Degeneration.". Frontiers in Neuroscience 11: 732. 2018. doi:10.3389/fnins.2017.00732. PMID 29354028.
- ↑ 69.0 69.1 "The white gene controls copulation success in Drosophila melanogaster". Scientific Reports 7 (1): 7712. August 2017. doi:10.1038/s41598-017-08155-y. PMID 28794482. Bibcode: 2017NatSR...7.7712X.
- ↑ "Gene:Dmel\y". The FlyBase Consortium. http://flybase.org/reports/FBgn0004034.html.
- ↑ "Reciprocal functions of the Drosophila yellow and ebony proteins in the development and evolution of pigment patterns". Development 129 (8): 1849–58. April 2002. doi:10.1242/dev.129.8.1849. PMID 11934851.
- ↑ 72.0 72.1 "Molecular analysis of the yellow gene (y) region of Drosophila melanogaster". Proceedings of the National Academy of Sciences of the United States of America 82 (21): 7369–73. November 1985. doi:10.1073/pnas.82.21.7369. PMID 3933004. Bibcode: 1985PNAS...82.7369B.
- ↑ "NCBI (National Center for Biotechnology Information) Genome Database". https://www.ncbi.nlm.nih.gov/genome/?term=drosophila%20melanogaster.
- ↑ "Ubiquitous selective constraints in the Drosophila genome revealed by a genome-wide interspecies comparison". Genome Research 16 (7): 875–84. July 2006. doi:10.1101/gr.5022906. PMID 16751341.
- ↑ "Origin and evolution of the Drosophila Y chromosome". Current Opinion in Genetics & Development 12 (6): 664–8. December 2002. doi:10.1016/S0959-437X(02)00356-8. PMID 12433579.
- ↑ "Functional and evolutionary implications of gene orthology". Nature Reviews. Genetics (Nature Portfolio) 14 (5): 360–366. May 2013. doi:10.1038/nrg3456. PMID 23552219.
- ↑ "Background on Comparative Genomic Analysis". US National Human Genome Research Institute. December 2002. https://www.genome.gov/10005835/.
- ↑ "A systematic analysis of human disease-associated gene sequences in Drosophila melanogaster". Genome Research 11 (6): 1114–25. June 2001. doi:10.1101/gr.169101. PMID 11381037.
- ↑ "Homophila: human disease gene cognates in Drosophila". Nucleic Acids Research 30 (1): 149–51. January 2002. doi:10.1093/nar/30.1.149. PMID 11752278.
- ↑ "Probing mechanisms that underlie human neurodegenerative diseases in Drosophila". Annual Review of Genetics 46: 371–96. 2012. doi:10.1146/annurev-genet-110711-155456. PMID 22974305.
- ↑ Fly Models of Human Diseases. Volume 121 of Current Topics in Developmental Biology. Academic Press. 2017. ISBN 978-0-12-802905-3. https://books.google.com/books?id=2zbZCgAAQBAJ.
- ↑ "Immunity in Drosophila melanogaster--from microbial recognition to whole-organism physiology". Nature Reviews. Immunology 14 (12): 796–810. December 2014. doi:10.1038/nri3763. PMID 25421701.
- ↑ "Drosophila melanogaster as a model to study drug addiction". Human Genetics 131 (6): 959–75. June 2012. doi:10.1007/s00439-012-1146-6. PMID 22350798.
- ↑ 84.0 84.1 "FlyMove--a new way to look at development of Drosophila". Trends in Genetics 19 (6): 310–1. June 2003. doi:10.1016/S0168-9525(03)00050-7. PMID 12801722.
- ↑ "Developmental plasticity and the origin of species differences". Proceedings of the National Academy of Sciences of the United States of America 102 (suppl 1): 6543–9. May 2005. doi:10.1073/pnas.0501844102. PMID 15851679. Bibcode: 2005PNAS..102.6543W.
- ↑ 86.0 86.1 86.2 "Behavioural effects of temperature on ectothermic animals: unifying thermal physiology and behavioural plasticity". Biological Reviews of the Cambridge Philosophical Society 92 (4): 1859–1876. November 2017. doi:10.1111/brv.12312. PMID 28980433.
- ↑ "Locomotor performance of Drosophila melanogaster: interactions among developmental and adult temperatures, age, and geography". Evolution; International Journal of Organic Evolution 55 (1): 205–9. January 2001. doi:10.1111/j.0014-3820.2001.tb01286.x. PMID 11263741.
- ↑ 88.0 88.1 88.2 "Bigger isn't always better: body size, developmental and parental temperature and male territorial success in Drosophila melanogaster" (in en). Animal Behaviour 49 (3): 671–677. 1995. doi:10.1016/0003-3472(95)80200-2. ISSN 0003-3472.
- ↑ "Developmental plasticity and stability in the tracheal networks supplying Drosophila flight muscle in response to rearing oxygen level". Journal of Insect Physiology. The limits of respiratory function: external and internal constraints on insect gas exchange 106 (Pt 3): 189–198. April 2018. doi:10.1016/j.jinsphys.2017.09.006. PMID 28927826.
- ↑ "Developmental plasticity of the locomotor activity rhythm of Drosophila melanogaster". Journal of Insect Physiology 48 (1): 25–32. January 2002. doi:10.1016/S0022-1910(01)00139-1. PMID 12770129.
- ↑ 91.0 91.1 91.2 91.3 "Within- and Between-Generation Effects of Temperature on the Morphology and Physiology of Drosophila Melanogaster". Evolution; International Journal of Organic Evolution 50 (3): 1205–1218. June 1996. doi:10.2307/2410661. PMID 28565273.
- ↑ 92.0 92.1 "Male sterility at extreme temperatures: a significant but neglected phenomenon for understanding Drosophila climatic adaptations". Journal of Evolutionary Biology 18 (4): 838–46. July 2005. doi:10.1111/j.1420-9101.2005.00914.x. PMID 16033555.
- ↑ "Body size and cell size in Drosophila: the developmental response to temperature". Journal of Insect Physiology 44 (11): 1081–1089. November 1998. doi:10.1016/S0022-1910(98)00061-4. PMID 12770407.
- ↑ "Cold rearing improves cold-flight performance in Drosophila via changes in wing morphology". The Journal of Experimental Biology 211 (Pt 13): 2116–22. July 2008. doi:10.1242/jeb.019422. PMID 18552301.
- ↑ 95.0 95.1 "Reversibility of developmental heat and cold plasticity is asymmetric and has long-lasting consequences for adult thermal tolerance". The Journal of Experimental Biology 219 (Pt 17): 2726–32. September 2016. doi:10.1242/jeb.143750. PMID 27353229.
- ↑ "Parental and developmental temperature effects on the thermal dependence of fitness in Drosophila melanogaster". Evolution; International Journal of Organic Evolution 55 (1): 209–14. January 2001. doi:10.1111/j.0014-3820.2001.tb01287.x. PMID 11263742.
- ↑ "Optimal temperature range of a plastic species, Drosophila simulans". The Journal of Animal Ecology 82 (3): 663–72. May 2013. doi:10.1111/1365-2656.12041. PMID 23360477. Bibcode: 2013JAnEc..82..663A.
- ↑ 98.0 98.1 "Different mechanisms underlie phenotypic plasticity and interspecific variation for a reproductive character in drosophilids (Insecta: Diptera)". Evolution; International Journal of Organic Evolution 54 (5): 1638–53. October 2000. doi:10.1111/j.0014-3820.2000.tb00708.x. PMID 11108591.
- ↑ "Drosophila is maximised by optimal developmental temperature". The Journal of Experimental Biology 222 (Pt 10): jeb202184. May 2019. doi:10.1242/jeb.202184. PMID 31064855.
- ↑ "Metabolic and functional characterization of effects of developmental temperature in Drosophila melanogaster". American Journal of Physiology. Regulatory, Integrative and Comparative Physiology 312 (2): R211–R222. February 2017. doi:10.1152/ajpregu.00268.2016. PMID 27927623.
- ↑ "Control of the adult reproductive potential by preimaginal thermal conditions: A study in Drosophila melanogaster". Oecologia 36 (3): 295–306. January 1978. doi:10.1007/BF00348055. PMID 28309916. Bibcode: 1978Oecol..36..295C.
- ↑ "The Sex Determination Gene transformer Regulates Male-Female Differences in Drosophila Body Size". PLOS Genetics 11 (12): e1005683. December 2015. doi:10.1371/journal.pgen.1005683. PMID 26710087.
- ↑ Developmental Biology (6th ed.). Sunderland (MA): Sinauer Associates; 2000. 2000. ISBN 978-0-87893-243-6. https://archive.org/details/developmentalbio00gilb.
- ↑ "The host defense of Drosophila melanogaster". Annual Review of Immunology 25: 697–743. 2007. doi:10.1146/annurev.immunol.25.022106.141615. PMID 17201680. http://infoscience.epfl.ch/record/151765/files/annurev2Eimmunol2E252E0221062E141615.pdf.
- ↑ "Comparative transcriptomics reveals CrebA as a novel regulator of infection tolerance in D. melanogaster". PLOS Pathogens 14 (2): e1006847. February 2018. doi:10.1371/journal.ppat.1006847. PMID 29394281.
- ↑ "The Toll and Imd pathways are the major regulators of the immune response in Drosophila". The EMBO Journal 21 (11): 2568–79. June 2002. doi:10.1093/emboj/21.11.2568. PMID 12032070.
- ↑ "Negative regulation by amidase PGRPs shapes the Drosophila antibacterial response and protects the fly from innocuous infection". Immunity 35 (5): 770–9. November 2011. doi:10.1016/j.immuni.2011.09.018. PMID 22118526. http://infoscience.epfl.ch/record/170470/files/1-s2.0-S1074761311004663-main.pdf.
- ↑ "Methods for the study of innate immunity in Drosophila melanogaster". Wiley Interdisciplinary Reviews. Developmental Biology 8 (5): e344. September 2019. doi:10.1002/wdev.344. PMID 30993906.
- ↑ "Drosophila melanogaster infection model". npj Microgravity 6 (1): 4. February 2020. doi:10.1038/s41526-019-0091-2. PMID 32047838.
- ↑ 110.0 110.1 "Thioester-containing proteins regulate the Toll pathway and play a role in Drosophila defence against microbial pathogens and parasitoid wasps". BMC Biology 15 (1): 79. September 2017. doi:10.1186/s12915-017-0408-0. PMID 28874153.
- ↑ "Drosophila melanogaster". eLife 5. November 2016. doi:10.7554/eLife.19662. PMID 27871362.
- ↑ "Cooperative regulation of the induction of the novel antibacterial Listericin by peptidoglycan recognition protein LE and the JAK-STAT pathway". The Journal of Biological Chemistry 285 (21): 15731–8. May 2010. doi:10.1074/jbc.M109.082115. PMID 20348097.
- ↑ "Drosophila as a model to study the role of blood cells in inflammation, innate immunity and cancer". Frontiers in Cellular and Infection Microbiology 3: 113. January 2014. doi:10.3389/fcimb.2013.00113. PMID 24409421.
- ↑ "Methods to study Drosophila immunity". Methods 68 (1): 116–28. June 2014. doi:10.1016/j.ymeth.2014.02.023. PMID 24631888. http://infoscience.epfl.ch/record/201328/files/1-s2.0-S104620231400067X-main.pdf.
- ↑ "Identification of lipoteichoic acid as a ligand for draper in the phagocytosis of Staphylococcus aureus by Drosophila hemocytes". Journal of Immunology 183 (11): 7451–60. December 2009. doi:10.4049/jimmunol.0901032. PMID 19890048.
- ↑ "The two origins of hemocytes in Drosophila". Development 130 (20): 4955–62. October 2003. doi:10.1242/dev.00702. PMID 12930778.
- ↑ "Adult Drosophila Lack Hematopoiesis but Rely on a Blood Cell Reservoir at the Respiratory Epithelia to Relay Infection Signals to Surrounding Tissues". Developmental Cell 51 (6): 787–803.e5. December 2019. doi:10.1016/j.devcel.2019.10.017. PMID 31735669.
- ↑ "Drosophila". eLife 8: e45061. July 2019. doi:10.7554/eLife.45061. PMID 31358113.
- ↑ "The claret mutant type of Drosophila simulans: a study of chromosome elimination and cell-lineage". Zeitschrift für Wissenschaftliche Zoologie 135: 323–356. 1929.
- ↑ "A new behavioral bioassay for an analysis of sexual attraction and pheromones in insects". The Journal of Experimental Zoology 192 (2): 271–5. May 1975. doi:10.1002/jez.1401920217. PMID 805823. Bibcode: 1975JEZ...192..271N. https://www.researchgate.net/publication/22347715.
- ↑ Biotechnology Fundamentals. CRC Press. 2011. p. 213. ISBN 978-1-4398-2009-4.
- ↑ "The 2017 Nobel Prize in Physiology or Medicine jointly to Jeffrey C. Hall, Michael Rosbash and Michael W. Young for their discoveries of molecular mechanisms controlling the circadian rhythm". Nobelprize.org. 2 October 2017. https://www.nobelprize.org/nobel_prizes/medicine/laureates/2017/press.html.
- ↑ "Distinct roles of TRP channels in auditory transduction and amplification in Drosophila". Neuron 77 (1): 115–28. January 2013. doi:10.1016/j.neuron.2012.11.030. PMID 23312520.
- ↑ "Sound response mediated by the TRP channels NOMPC, NANCHUNG, and INACTIVE in chordotonal organs of Drosophila larvae". Proceedings of the National Academy of Sciences of the United States of America 110 (33): 13612–7. August 2013. doi:10.1073/pnas.1312477110. PMID 23898199. Bibcode: 2013PNAS..11013612Z.
- ↑ "Homosexuality Turned On and Off in Fruit Flies"
- ↑ "A neural data structure for novelty detection". Proceedings of the National Academy of Sciences of the United States of America 115 (51): 13093–13098. December 2018. doi:10.1073/pnas.1814448115. PMID 30509984. Bibcode: 2018PNAS..11513093D.
- ↑ "Genetics and neurobiology of aggression in Drosophila". Fly 6 (1): 35–48. 2012-01-01. doi:10.4161/fly.19249. PMID 22513455.
- ↑ "Drosophila Using a Screen for Wing Damage". Genetics 208 (1): 273–282. January 2018. doi:10.1534/genetics.117.300292. PMID 29109180.
- ↑ 129.0 129.1 129.2 "Drosophila aggression". Proceedings of the National Academy of Sciences of the United States of America 114 (8): 1958–1963. February 2017. doi:10.1073/pnas.1605946114. PMID 28115690.
- ↑ 130.0 130.1 "How Drosophila Detect Volatile Pheromones: Signaling, Circuits, and Behavior". Neurobiology of Chemical Communication. Frontiers in Neuroscience. CRC Press/Taylor & Francis. 2014. ISBN 978-1-4665-5341-5. http://www.ncbi.nlm.nih.gov/books/NBK200999/. Retrieved 2019-05-30.
- ↑ "Drosophila melanogaster females restore their attractiveness after mating by removing male anti-aphrodisiac pheromones". Nature Communications 7 (1): 12322. August 2016. doi:10.1038/ncomms12322. PMID 27484362. Bibcode: 2016NatCo...712322L.
- ↑ "Hierarchical chemosensory regulation of male-male social interactions in Drosophila". Nature Neuroscience 14 (6): 757–62. June 2011. doi:10.1038/nn.2800. PMID 21516101.
- ↑ 133.0 133.1 133.2 "How food controls aggression in Drosophila". PLOS ONE 9 (8): e105626. 2014-08-27. doi:10.1371/journal.pone.0105626. PMID 25162609. Bibcode: 2014PLoSO...9j5626L.
- ↑ "Interaction between sleep and metabolism in Drosophila with altered octopamine signaling". The Journal of Biological Chemistry 287 (39): 32406–14. September 2012. doi:10.1074/jbc.M112.360875. PMID 22829591.
- ↑ 135.0 135.1 135.2 "Sleep deprivation suppresses aggression in Drosophila". eLife 4: e07643. July 2015. doi:10.7554/eLife.07643. PMID 26216041.
- ↑ "Visual transduction in Drosophila". Nature 413 (6852): 186–93. September 2001. doi:10.1038/35093002. PMID 11557987. Bibcode: 2001Natur.413..186H.
- ↑ 137.0 137.1 137.2 137.3 "The spectral sensitivity of Drosophila photoreceptors". Scientific Reports 10 (1): 18242. October 2020. doi:10.1038/s41598-020-74742-1. PMID 33106518. Bibcode: 2020NatSR..1018242S.
Material was copied and adapted from this source, which is available under a Creative Commons Attribution 4.0 International License.
- ↑ "Phylogenomics of Opsin Genes in Diptera Reveals Lineage-Specific Events and Contrasting Evolutionary Dynamics in Anopheles and Drosophila". Genome Biology and Evolution 13 (8): evab170. August 2021. doi:10.1093/gbe/evab170. PMID 34270718.
- ↑ "Genetic dissection of the photoreceptor system in the compound eye of Drosophila melanogaster". The Journal of Physiology 256 (2): 415–439. April 1976. doi:10.1113/jphysiol.1976.sp011331. PMID 16992509.
- ↑ 140.0 140.1 "Drosophila rhodopsin: photochemistry, extraction and differences in the norp AP12 phototransduction mutant". Biochemical and Biophysical Research Communications 59 (3): 960–966. August 1974. doi:10.1016/s0006-291x(74)80073-2. PMID 4213042.
- ↑ "Characteristics of Drosophila rhodopsin in wild-type and norpA vision transduction mutants". The Journal of General Physiology 72 (5): 717–732. November 1978. doi:10.1085/jgp.72.5.717. PMID 105082.
- ↑ 142.0 142.1 142.2 142.3 142.4 142.5 142.6 "Blue- and green-absorbing visual pigments of Drosophila: ectopic expression and physiological characterization of the R8 photoreceptor cell-specific Rh5 and Rh6 rhodopsins". The Journal of Neuroscience 19 (24): 10716–10726. December 1999. doi:10.1523/jneurosci.19-24-10716.1999. PMID 10594055.
- ↑ "Evidence for a sensitising pigment in fly photoreceptors". Nature 269 (5627): 386–390. September 1977. doi:10.1038/269386a0. PMID 909585. Bibcode: 1977Natur.269..386K.
- ↑ "The contribution of a sensitizing pigment to the photosensitivity spectra of fly rhodopsin and metarhodopsin". The Journal of General Physiology 73 (5): 517–540. May 1979. doi:10.1085/jgp.73.5.517. PMID 458418.
- ↑ 145.0 145.1 "A photostable pigment within the rhabdomere of fly photoreceptors no. 7". Journal of Comparative Physiology A 125 (3): 275–284. 1978. doi:10.1007/BF00656606.
- ↑ "Photostable pigments within the membrane of photoreceptors and their possible role". Biophysics of Structure and Mechanism 3 (2): 191–194. June 1977. doi:10.1007/BF00535818. PMID 890056.
- ↑ "A rhodopsin gene expressed in photoreceptor cell R7 of the Drosophila eye: homologies with other signal-transducing molecules". The Journal of Neuroscience 7 (5): 1550–1557. May 1987. doi:10.1523/jneurosci.07-05-01550.1987. PMID 2437266.
- ↑ 148.0 148.1 148.2 "Identification of a novel Drosophila opsin reveals specific patterning of the R7 and R8 photoreceptor cells". Neuron 17 (6): 1101–1115. December 1996. doi:10.1016/s0896-6273(00)80243-3. PMID 8982159.
- ↑ 149.0 149.1 149.2 149.3 "Ectopic expression of ultraviolet-rhodopsins in the blue photoreceptor cells of Drosophila: visual physiology and photochemistry of transgenic animals". The Journal of Neuroscience 12 (10): 3862–3868. October 1992. doi:10.1523/jneurosci.12-10-03862.1992. PMID 1403087.
- ↑ "A second opsin gene expressed in the ultraviolet-sensitive R7 photoreceptor cells of Drosophila melanogaster". The Journal of Neuroscience 7 (5): 1558–1566. May 1987. doi:10.1523/JNEUROSCI.07-05-01558.1987. PMID 2952772.
- ↑ "Molecular cloning of Drosophila Rh6 rhodopsin: the visual pigment of a subset of R8 photoreceptor cells". FEBS Letters 406 (1–2): 6–10. April 1997. doi:10.1016/s0014-5793(97)00210-x. PMID 9109375.
- ↑ "Transcript localization of four opsin genes in the three visual organs of Drosophila; RH2 is ocellus specific". Nature 333 (6175): 779–782. June 1988. doi:10.1038/333779a0. PMID 2968518. Bibcode: 1988Natur.333..779P.
- ↑ 153.0 153.1 "Targeted misexpression of a Drosophila opsin gene leads to altered visual function". Nature 333 (6175): 737–741. June 1988. doi:10.1038/333737a0. PMID 2455230. Bibcode: 1988Natur.333..737F.
- ↑ "Drosophila melanogaster rhodopsin Rh7 is a UV-to-visible light sensor with an extraordinarily broad absorption spectrum". Scientific Reports 7 (1): 7349. August 2017. doi:10.1038/s41598-017-07461-9. PMID 28779161. Bibcode: 2017NatSR...7.7349S.
- ↑ "A rhodopsin in the brain functions in circadian photoentrainment in Drosophila". Nature 545 (7654): 340–344. May 2017. doi:10.1038/nature22325. PMID 28489826. Bibcode: 2017Natur.545..340N.
- ↑ "The Chromophore of the Visual Pigment in Some Insect Orders". Zeitschrift für Naturforschung C 39 (1–2): 196–197. 1 February 1984. doi:10.1515/znc-1984-1-236.
- ↑ "Chemical identity of the chromophores of fly visual pigment". Naturwissenschaften 71 (4): 211–213. April 1984. doi:10.1007/BF00490436. Bibcode: 1984NW.....71..211V.
- ↑ "The Gluopsins: Opsins without the Retinal Binding Lysine". Cells 11 (15): 2441. August 2022. doi:10.3390/cells11152441. PMID 35954284.
- ↑ "Chromophore-Independent Roles of Opsin Apoproteins in Drosophila Mechanoreceptors". Current Biology 29 (17): 2961–2969.e4. September 2019. doi:10.1016/j.cub.2019.07.036. PMID 31447373.
- ↑ 160.0 160.1 "Functions of Opsins in Drosophila Taste". Current Biology 30 (8): 1367–1379.e6. April 2020. doi:10.1016/j.cub.2020.01.068. PMID 32243853.
- ↑ "Melanopsin triggers the release of internal calcium stores in response to light". Photochemistry and Photobiology 83 (2): 273–279. March 2007. doi:10.1562/2006-07-11-RA-964. PMID 16961436.
- ↑ 162.0 162.1 "Normal phototransduction in Drosophila photoreceptors lacking an InsP(3) receptor gene". Molecular and Cellular Neurosciences 15 (5): 429–45. May 2000. doi:10.1006/mcne.2000.0846. PMID 10833300.
- ↑ "Phototransduction in Drosophila". Current Opinion in Neurobiology 34: 37–45. October 2015. doi:10.1016/j.conb.2015.01.008. PMID 25638280. https://www.repository.cam.ac.uk/handle/1810/247230.
- ↑ "Light activation, adaptation, and cell survival functions of the Na+/Ca2+ exchanger CalX". Neuron 45 (3): 367–78. February 2005. doi:10.1016/j.neuron.2004.12.046. PMID 15694324.
- ↑ "The Drosophila standard brain". Current Biology 12 (3): 227–31. February 2002. doi:10.1016/S0960-9822(02)00656-5. PMID 11839276.
- ↑ "Hierarchical organization and postural facilitation: rules for grooming in flies". Animal Behaviour 24 (4): 739–755. 1976. doi:10.1016/S0003-3472(76)80003-6.
- ↑ 167.0 167.1 167.2 "Behavioural hierarchies". Trends in Neurosciences 2 (2): 5–7. 1979. doi:10.1016/0166-2236(79)90003-1.
- ↑ 168.0 168.1 "A suppression hierarchy among competing motor programs drives sequential grooming in Drosophila". eLife 3: e02951. August 2014. doi:10.7554/eLife.02951. PMID 25139955.
- ↑ "DeepLabCut: markerless pose estimation of user-defined body parts with deep learning". Nature Neuroscience 21 (9): 1281–1289. September 2018. doi:10.1038/s41593-018-0209-y. PMID 30127430.
- ↑ "Coordination of legs during straight walking and turning in Drosophila melanogaster". Journal of Comparative Physiology A 167 (3): 403–12. August 1990. doi:10.1007/BF00192575. PMID 2121965.
- ↑ "Drosophila". eLife 8. June 2019. doi:10.7554/eLife.46409. PMID 31250807.
- ↑ "Inter-leg coordination in the control of walking speed in Drosophila". The Journal of Experimental Biology 216 (Pt 3): 480–91. February 2013. doi:10.1242/jeb.078139. PMID 23038731.
- ↑ "Quantification of gait parameters in freely walking wild type and sensory deprived Drosophila melanogaster". eLife 2: e00231. January 2013. doi:10.7554/eLife.00231. PMID 23326642.
- ↑ "Drosophila". The Journal of Experimental Biology 221 (Pt 22): jeb189142. November 2018. doi:10.1242/jeb.189142. PMID 30274987.
- ↑ "Body size and the neural control of movement". Current Biology 22 (9): R318-22. May 2012. doi:10.1016/j.cub.2012.02.048. PMID 22575473.
- ↑ 176.0 176.1 176.2 "The aerodynamics of free-flight maneuvers in Drosophila". Science 300 (5618): 495–8. April 2003. doi:10.1126/science.1081944. PMID 12702878. Bibcode: 2003Sci...300..495F. http://www.ini.unizh.ch/~pfmjv/InsectCognition/science_300_495.fly-flight.pdf.
- ↑ "Turning behaviour depends on frictional damping in the fruit fly Drosophila". The Journal of Experimental Biology 210 (Pt 24): 4319–34. December 2007. doi:10.1242/jeb.010389. PMID 18055621.
- ↑ "Three-dimensional reconstruction of brain-wide wiring networks in Drosophila at single-cell resolution". Current Biology 21 (1): 1–11. January 2011. doi:10.1016/j.cub.2010.11.056. PMID 21129968.
- ↑ "FlyCircuit - A Database of Drosophila Brain Neurons". http://flycircuit.tw/.
- ↑ "Synaptic organization of columnar elements in the lamina of the wild type in Drosophila melanogaster". The Journal of Comparative Neurology 305 (2): 232–63. March 1991. doi:10.1002/cne.903050206. PMID 1902848.
- ↑ "Wiring economy and volume exclusion determine neuronal placement in the Drosophila brain". Current Biology 21 (23): 2000–5. December 2011. doi:10.1016/j.cub.2011.10.022. PMID 22119527.
- ↑ "A visual motion detection circuit suggested by Drosophila connectomics". Nature 500 (7461): 175–81. August 2013. doi:10.1038/nature12450. PMID 23925240. Bibcode: 2013Natur.500..175T.
- ↑ "Drosophila brain". eLife 6: e26975. July 2017. doi:10.7554/eLife.26975. PMID 28718765.
- ↑ "Entire Fruit Fly Brain Imaged with Electron Microscopy" (in en). https://www.the-scientist.com/the-scientist/entire-fruit-fly-brain-imaged-with-electron-microscopy-31449.
- ↑ "A Complete Electron Microscopy Volume of the Brain of Adult Drosophila melanogaster". Cell 174 (3): 730–743.e22. July 2018. doi:10.1016/j.cell.2018.06.019. PMID 30033368.
- ↑ "A connectome of the adult Drosophila central brain". bioRxiv (Cold Spring Harbor Laboratory): 2020.01.21.911859. 2020. doi:10.1101/2020.01.21.911859.
- ↑ "Analysis tools for connectomics". HHMI. https://neuprint.janelia.org.
- ↑ Phelps, Jasper S.; Hildebrand, David Grant Colburn; Graham, Brett J.; Kuan, Aaron T.; Thomas, Logan A.; Nguyen, Tri M.; Buhmann, Julia; Azevedo, Anthony W. et al. (2021-02-02). "Reconstruction of motor control circuits in adult Drosophila using automated transmission electron microscopy" (in en). Cell 184 (3): 759–774.e18. doi:10.1016/j.cell.2020.12.013. PMID 33400916.
- ↑ "A Connectome and Analysis of the Adult Drosophila Central Brain". bioRxiv (Cold Spring Harbor) 9. 2020. doi:10.1101/2020.04.07.030213. PMID 32880371.
- ↑ Popovych, Sergiy; Macrina, Thomas; Kemnitz, Nico; Castro, Manuel; Nehoran, Barak; Jia, Zhen; Bae, J. Alexander; Mitchell, Eric; Mu, Shang; Trautman, Eric T.; Saalfeld, Stephan; Li, Kai; Seung, Sebastian (2022-03-27). "Petascale pipeline for precise alignment of images from serial section electron microscopy". bioRxiv 10.1101/2022.03.25.485816.
- ↑ Winding, Michael; Pedigo, Benjamin D.; Barnes, Christopher L.; Patsolic, Heather G.; Park, Youngser; Kazimiers, Tom; Fushiki, Akira; Andrade, Ingrid V. et al. (2023-03-10). "The connectome of an insect brain" (in en). Science 379 (6636): eadd9330. doi:10.1126/science.add9330. PMID 36893230.
- ↑ "Non pest species". Plant Health Australia. http://preventfruitfly.com.au/why-is-fruit-fly-a-problem/non-pest-species.
- ↑ "Fruit Flies: A Case Of Mistaken Identity". Australian Museum. February 5, 2014. https://australianmuseum.net.au/blogpost/science/fruit-flies-mistaken-identity.
Further reading
- Lords of the Fly: Drosophila genetics and the experimental life. Chicago: University of Chicago Press. 1994. ISBN 978-0-226-45063-6. https://archive.org/details/lordsofflydrosop0000kohl.
- Developmental Biology. (6th ed.). Sunderland (MA): Sinauer Associates; 2000. 2000. ISBN 978-0-87893-243-6. https://archive.org/details/developmentalbio00gilb.
- "Fruit flies on the front line: the translational impact of Drosophila". Disease Models & Mechanisms 9 (3): 229–31. March 2016. doi:10.1242/dmm.024810. PMID 26935101.
- "Row over fruit fly Drosophila melanogaster name bugs scientists". The Times (The Australian). April 8, 2010. http://www.theaustralian.com.au/news/world/row-over-fruit-fly-drosophila-melanogaster-name-bugs-scientists/news-story/d95720128e4eb89d00d1917951791cfa.
External links
![]() |
Wikimedia Commons has media related to Drosophila melanogaster. |
- "A quick and simple introduction to Drosophila melanogaster". Drosophila Virtual Library. http://ceolas.org/VL/fly/intro.html.
- "Drosophila Genomics Resource Center" – collects, maintains and distributes Drosophila DNA clones and cell lines.
- "Bloomington Drosophila Stock Center" – collects, maintains and distributes Drosophila melanogaster strains for research
- "FlyBase—A Database of Drosophila Genes & Genomes". http://flybase.net/.
- "NCBI Map Viewer – Drosophila melanogaster". https://www.ncbi.nlm.nih.gov/mapview/map_search.cgi?taxid=7227.
- "Drosophila Virtual Library". http://www.ceolas.org/VL/fly/.
- "The Berkeley Drosophila Genome Project". http://www.fruitfly.org/.
- "FlyMove". http://flymove.uni-muenster.de/. – video resources for Drosophila development
- "Drosophila Nomenclature—naming of genes". http://www.flynome.com/index.html.
- View the Fruitfly genome on Ensembl
- View the dm6 genome assembly in the UCSC Genome Browser.
- Manchester Fly Facility – for the public from the University of Manchester
- The droso4schools website with school-relevant resources about Drosophila
- Part 1 of the "Small fly: BIG impact" educational videos explaining the history and importance of the model organism Drosophila.
- Part 2 of the "Small fly: BIG impact" educational videos explaining how research is carried out in Drosophila.
- "Inside the Fly Lab"—broadcast by WGBH and PBS, in the program series Curious, January 2008.
- "How a Fly Detects Poison" —WhyFiles.org article describes how the fruit fly tastes a larva-killing chemical in food.
Wikidata ☰ Q130888 entry
![]() | Original source: https://en.wikipedia.org/wiki/Drosophila melanogaster.
Read more |