Biology:C3 carbon fixation
C3 carbon fixation is the most common of three metabolic pathways for carbon fixation in photosynthesis, the other two being C4 and CAM. This process converts carbon dioxide and ribulose bisphosphate (RuBP, a 5-carbon sugar) into two molecules of 3-phosphoglycerate through the following reaction:
- CO2 + H2O + RuBP → (2) 3-phosphoglycerate
This reaction was first discovered by Melvin Calvin, Andrew Benson and James Bassham in 1950.[1] C3 carbon fixation occurs in all plants as the first step of the Calvin–Benson cycle. (In C4 and CAM plants, carbon dioxide is drawn out of malate and into this reaction rather than directly from the air.)
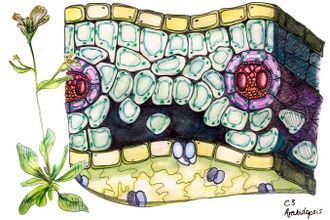
Plants that survive solely on C3 fixation (C3 plants) tend to thrive in areas where sunlight intensity is moderate, temperatures are moderate, carbon dioxide concentrations are around 200 ppm or higher,[2] and groundwater is plentiful. The C3 plants, originating during Mesozoic and Paleozoic eras, predate the C4 plants and still represent approximately 95% of Earth's plant biomass, including important food crops such as rice, wheat, soybeans and barley.
C3 plants cannot grow in very hot areas at today's atmospheric CO2 level (significantly depleted during hundreds of millions of years from above 5000 ppm) because RuBisCO incorporates more oxygen into RuBP as temperatures increase. This leads to photorespiration (also known as the oxidative photosynthetic carbon cycle, or C2 photosynthesis), which leads to a net loss of carbon and nitrogen from the plant and can therefore limit growth.
C3 plants lose up to 97% of the water taken up through their roots by transpiration.[3] In dry areas, C3 plants shut their stomata to reduce water loss, but this stops CO
2 from entering the leaves and therefore reduces the concentration of CO
2 in the leaves. This lowers the CO
2:O2 ratio and therefore also increases photorespiration. C4 and CAM plants have adaptations that allow them to survive in hot and dry areas, and they can therefore out-compete C3 plants in these areas.
The isotopic signature of C3 plants shows higher degree of 13C depletion than the C4 plants, due to variation in fractionation of carbon isotopes in oxygenic photosynthesis across plant types. Specifically, C3 plants do not have PEP carboxylase like C4 plants, allowing them to only utilize ribulose-1,5-bisphosphate carboxylase (Rubisco) to fix CO
2 through the Calvin cycle. The enzyme Rubisco largely discriminates against carbon isotopes, evolving to only bind to 12C isotope compared to 13C (the heavier isotope), attributing to why there's a low 13C depletion seen in C3 plants compared to C4 plants especially since the C4 pathway uses PEP carboxylase in addition to Rubisco.[4]
Variations
Not all C3 carbon fixation pathways operate at the same efficiency.
Refixation
Bamboos and the related rice have an improved C3 efficiency. This improvement might be due to its ability to recapture CO2 produced during photorespiration, a behavior termed "carbon refixation". These plants achieve refixation by growing chloroplast extensions called "stromules" around the stroma in mesophyll cells, so that any photorespired CO2 from the mitochondria has to pass through the RuBisCO-filled chloroplast.[5]
Refixation is also performed by a wide variety of plants. The common approach involving growing a bigger bundle sheath leads down to C2 photosynthesis.[6]
Synthetic glycolate pathway
C3 carbon fixation is prone to photorespiration (PR) during dehydration, accumulating toxic glycolate products. In the 2000s scientists used computer simulation combined with an optimization algorithm to figure out what parts of the metabolic pathway may be tuned to improve photosynthesis. According to simulation, improving glycolate metabolism would help significantly to reduce photorespiration.[7][8]
Instead of optimizing specific enzymes on the PR pathway for glycolate degradation, South et al. decided to bypass PR altogether. In 2019, they transferred Chlamydomonas reinhardtii glycolate dehydrogenase and Cucurbita maxima malate synthase into the chloroplast of tobacco (a C3 model organism). These enzymes, plus the chloroplast's own, create a catabolic cycle: acetyl-CoA combines with glyoxylate to form malate, which is then split into pyruvate and CO2; the former in turn splits into acetyl-CoA and CO2. By forgoing all transport among organelles, all the CO2 released will go into increasing the CO2 concentration in the chloroplast, helping with refixation. The end result is 24% more biomass. An alternative using E. coli glycerate pathway produced a smaller improvement of 13%. They are now working on moving this optimization into other C3 crops like wheat.[9]
References
- ↑ "Forty years of photosynthesis and related activities". Interdisciplinary Science Reviews 22 (2): 138–148. 1997. doi:10.1179/isr.1997.22.2.138. Bibcode: 1997ISRv...22..138C.
- ↑ "Respiration". Encyclopedia of Earth. Washington, D.C.: National Council for Science and the Environment.. 2011. http://www.eoearth.org/article/Respiration?topic=74360.
- ↑ "Roots: evolutionary origins and biogeochemical significance". Journal of Experimental Botany 52 (Spec Issue): 381–401. March 2001. doi:10.1093/jexbot/52.suppl_1.381. PMID 11326045.
- ↑ "Carbon isotope discrimination as a diagnostic tool for C4 photosynthesis in C3-C4 intermediate species". Journal of Experimental Botany 67 (10): 3109–21. May 2016. doi:10.1093/jxb/erv555. PMID 26862154.
- ↑ Peixoto, Murilo M.; Sage, Tammy L.; Busch, Florian A.; Pacheco, Haryel D. N.; Moraes, Moemy G.; Portes, Tomás A.; Almeida, Rogério A.; Graciano-Ribeiro, Dalva et al. (27 March 2021). "Elevated efficiency of C3 photosynthesis in bamboo grasses: A possible consequence of enhanced refixation of photorespired CO2". GCB Bioenergy 13 (6): 941–954. doi:10.1111/gcbb.12819.
- ↑ Sage, Rowan F.; Khoshravesh, Roxana; Sage, Tammy L. (1 July 2014). "From proto-Kranz to C4 Kranz: building the bridge to C4 photosynthesis". Journal of Experimental Botany 65 (13): 3341–3356. doi:10.1093/jxb/eru180. PMID 24803502.
- ↑ "Optimizing the distribution of resources between enzymes of carbon metabolism can dramatically increase photosynthetic rate: a numerical simulation using an evolutionary algorithm". Plant Physiology 145 (2): 513–26. October 2007. doi:10.1104/pp.107.103713. PMID 17720759.
- ↑ "Analysis and Optimization of C3 Photosynthetic Carbon Metabolism". 2010 IEEE International Conference on BioInformatics and BioEngineering. Philadelphia, PA, USA: IEEE. 2010. pp. 44–51. doi:10.1109/BIBE.2010.17. ISBN 978-1-4244-7494-3. https://www.researchgate.net/publication/242452848.
- ↑ "Synthetic glycolate metabolism pathways stimulate crop growth and productivity in the field". Science 363 (6422): eaat9077. January 2019. doi:10.1126/science.aat9077. PMID 30606819.
![]() | Original source: https://en.wikipedia.org/wiki/C3 carbon fixation.
Read more |