Earth:Stratification (water)
Stratification is defined as the separation of water in layers based on a specific quantity. Two main types of stratification of water are uniform and layered stratification. Layered stratification occurs in all of the ocean basins. The stratified layers act as a barrier to the mixing of water, which can impact the exchange of heat, carbon, oxygen and other nutrients.[1] Due to upwelling and downwelling, which are both wind-driven, mixing of different layers can occur by means of the rise of cold nutrient-rich and warm water, respectively. Generally, the layers are based on the density of water. Intuitively, heavier, and hence denser, water is located below the lighter water, representing a stable stratification. An example of a layer in the ocean is the pycnocline, which is defined as a layer in the ocean where the change in density is relatively large compared to the other layers in the ocean. The thickness of the thermocline is not constant everywhere, but depends on a variety of variables. Between 1960 and 2018, upper ocean stratification has increased between 0.7-1.2% per decade.[1] An increase in stratification means that the differences in density of the layers in the oceans increase, leading to for example larger mixing barriers.
Density of water in the oceans
The density of water in the ocean, which is defined as mass per unit of volume, has a complicated dependence on temperature ([math]\displaystyle{ T }[/math]), salinity ([math]\displaystyle{ S }[/math]) and pressure ([math]\displaystyle{ p }[/math]) (or equivalently depth) and is denoted as [math]\displaystyle{ \rho(S, T, p) }[/math]. The dependence on pressure is not significant, since seawater is almost perfectly incompressible.[2] A change in the temperature of the water impacts on the distance between water parcels directly. When the temperature of the water increases, the distance between water parcels will increase and hence the density will decrease. A change in salinity induces an opposite reaction. Salinity is a measure of the mass of dissolved solids, which consist mainly of salt. Hence, increasing the salinity will increase the density. Just like the pycnocline defines the layer with a fast change in density, similar layers can be defined for a fast change in temperature and salinity: the thermocline and the halocline. Since the density depends on both the temperature and the salinity, the pycno-, thermo-, and halocline have a similar shape. The difference is that the density increases with depth, whereas the salinity and temperature decrease with depth.
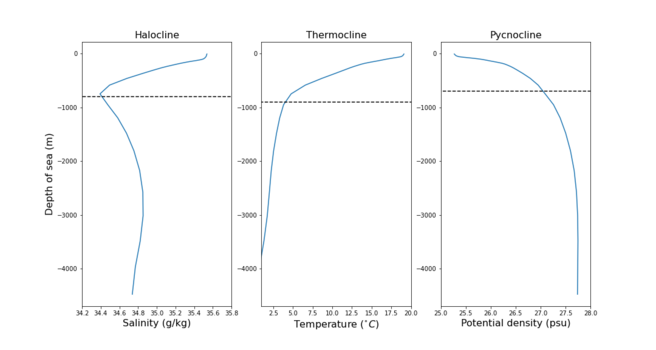
In the ocean, a specific range of temperature and salinity occurs. Using the GODAS Data,[3] a temperature-salinity plot can show the possibilities and occurrences of the different combinations of salinity and potential temperature.
![]() Potential temperature - salinity plot. This plot was generated using the GODAS Data[3] of 2020. |
![]() Occurrences of combinations of potential temperature and salinity in the ocean. This plot was generated using the GODAS Data[3] of 2020. |
The exact formula of density in the ocean can be defined by the UNESCO formula. According to this formula, the density of ocean water can be determined as follows:[4] [math]\displaystyle{ \rho = \frac{\rho(S, T, 0)}{1-\frac{p}{K(S, T, p)}}. }[/math]The terms in this formula, density when the pressure is zero, [math]\displaystyle{ \rho(S, T, 0) }[/math], and a term involving the compressibility of water, [math]\displaystyle{ K(S, T, p) }[/math], are both heavily dependent on the temperature and less dependent on the salinity:[math]\displaystyle{ \begin{align}\rho(S, T, 0) = \rho_{SMOW} + B_1S + C_1S^{1.5} + d_0S^2, &\qquad K(S, T, p) =K(S, T, 0) + A_1p + B_2p^2, \end{align} }[/math]
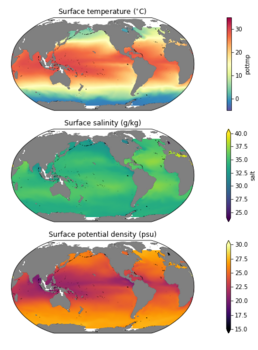
with:[math]\displaystyle{ \begin{align} {} & \rho_{SMOW} = a_0 + a_1T + a_2T^2 + a_3T^3 + a_4T^4 + a_5T^5, \\ {} & B_1 = b_0 + b_1T + b_2T^2 + b_3T^3 + b_4T^4, \\ {} & C_1 = c_0 + c_1T + c_2T^2, \\ \end{align} }[/math]and[math]\displaystyle{ \begin{align} {} & K(S, T, 0) = K_w + F_1S + G_1S^{1.5}, \\ {} & K_w = e_0 + e_1T + e_2T^2 + e_3T^3 + e^4T^4, \\ {} & F_1 = f_0 + f_1T + f_1T + f_2T^2 + f_3T^3, \\ {} & G_1 = g_0 + g_1T + g_2T^2, \\ {} & A_1 = A_w + (i_0 + i_1T + i_2T^2)S + j_0S^{1.5}, \\ {} & A_w = h_0 + h_1T + h_2T^2 + h_3T^3, \\ {} & B_2 = B_w + (m_0 + m_1T + m_2T^2)S), \\ {} & B_w = k_0 + k_1T + k_2T^2. \end{align} }[/math]In these formulas, all of the small letters, [math]\displaystyle{ a_i, b_i, c_i, d_0, e_i, f_i, g_i, i_i, j_0, h_i, m_i }[/math] and [math]\displaystyle{ k_i }[/math] are constants that are defined in Appendix A of a book on Internal Gravity Waves, published in 2015.[4]
The density depends more on the temperature than on the salinity, as can be deduced from the exact formula and can be shown in plots using the GODAS Data.[3] In the plots regarding surface temperature, salinity and density, it can be seen that locations with the coldest water, at the poles, are also the locations with the highest densities. The regions with the highest salinity, on the other hand, are not the regions with the highest density, meaning that temperature contributes mostly to the density in the oceans. A specific example is the Arabic Sea.
Ocean stratification
Ocean stratification can be defined and quantified by the change in density with depth. It is not uncommon to use the Buoyancy frequency, or sometimes called the Brunt-Väisälä frequency as direct representation of stratification in combination with observations on temperature and salinity. The Buoyancy frequency, [math]\displaystyle{ N }[/math], representing the intrinsic frequency of internal waves,[1] is defined as follows:[math]\displaystyle{ N^2 = \frac{-g}{\rho_0}\frac{\partial\rho}{\partial z}. }[/math]Here, [math]\displaystyle{ g }[/math] is the gravitational constant, [math]\displaystyle{ \rho_0 }[/math] is a reference density and [math]\displaystyle{ \rho }[/math] is the potential density depending on temperature and salinity as discussed earlier. Water is considered to have a stable stratification for [math]\displaystyle{ \partial\rho/\partial z\lt 0 }[/math], leading to a real value of [math]\displaystyle{ N }[/math]. The ocean is typically stable and the corresponding [math]\displaystyle{ N }[/math]-values in the ocean lie between approximately [math]\displaystyle{ 10^{-4} }[/math] in the abyssal ocean and [math]\displaystyle{ 10^{-3} }[/math] in the upper parts of the ocean. The Buoyancy period is defined as [math]\displaystyle{ 1/N }[/math]. Corresponding to the previous values, this period typically takes values between approximately 10 and 100 minutes.[5] In some parts of the ocean unstable stratification appears, leading to convection.
If the stratification in a water column increases, implying an increase of the value [math]\displaystyle{ N^2 }[/math], turbulent mixing and hence the eddy viscosity will decrease.[6] Furthermore, an increase of [math]\displaystyle{ N^2 }[/math], implies an increase of [math]\displaystyle{ |\partial\rho/\partial z| }[/math], meaning that the difference in densities in this water column increase as well. Throughout the year, the oceanic stratification is not constant, since the stratification depends on density, and therefore on temperature and salinity. The interannual fluctuations in tropical Pacific Ocean stratification are dominated by El Niño, which can be linked with the strong variations in the thermocline depth in the eastern equatorial Pacific.[1] Furthermore, tropical storms are sensitive to the conditions on the stratification and hence on its change.[7]
Observations on change in stratification in the oceans
In the last few decades, the stratification in all of the ocean basins has increased. Furthermore, the southern oceans (south of 30°S) experienced the strongest rate of stratification since 1960, followed by the Pacific Ocean, the Atlantic Ocean, and the Indian Ocean.[1] When the upper ocean becomes more stratified, the mixed layer of surface water with homogeneous temperature may get shallower, but projected changes to mixed-layer depth by the end of the 21st century remain contested.[8] The regions with the currently deepest mixed layers are associated with the greatest mixed layer shoaling, particularly the North Atlantic and Southern Ocean basin.[8] By looking at the GODAS Data[3] provided by the NOAA/OAR/ESRL PSL, the Buoyancy frequencies can be found from January 1980 up to and including March 2021. Since a change in stratification is mostly visible in the upper 500 meters of the ocean, very specific data is necessary in order to see this in a plot. The resulting plots from the GODAS Data might indicate that there is also a decrease in stratification looking at the differences of the stratification between the years 1980, 2000 and 2020. It is possible to see that the change in stratification is indeed the biggest in the first 500 meters of the ocean. From approximately 1000 meters into the ocean, the stratification converges toward a stable value and the change in stratification becomes almost non-existent.
![]() Annual and latitudinal means of [math]\displaystyle{ N^2 }[/math] for different ocean basins. This plot was generated using the GODAS Data[3] of 1980, 2000 and 2020. |
![]() Change in annual and latitudinal means of [math]\displaystyle{ N^2 }[/math] for different ocean basins. This plot was generated using the GODAS Data[3] of 1980, 2000 and 2020. |
In many scientific articles, magazines and blogs, it is claimed that the stratification has increased in all of the ocean basins (e.g in Ecomagazine.com[9] and NCAR & UCAR News [10]). In the figure below, the trends of the change in stratification in all of the ocean basins have been plotted.[1] This data shows that over the years the stratification has drastically increased. The changes in stratification are greatest in the Southern Ocean, followed by the Pacific Ocean. In the Pacific Ocean, the increase of stratification in the eastern equatorial has found to be greater than in the western equatorial.[1] This is likely to be linked to the weakening of the trade winds and reduced upwelling in the eastern Pacific, which can be explained by the weakening of the Walker circulation.[1]

Change in ocean stratification: causes and consequences
Temperature and mixing
The change in temperature dominates the increasing stratification, while salinity only plays a role locally.[1] The ocean has an extraordinary ability of storing and transporting large amounts of heat, carbon and fresh water.[11] Even though approximately 70% of the Earth's surface consists of water, more than 75% of the water exchange between the Earth's surface and the atmosphere occurs over the oceans. The ocean absorbs part of the energy from sunlight as heat and is initially absorbed by the surface.[12] Eventually a part of this heat also spreads to deeper water. Greenhouse gases absorb extra energy from the sun, which is again absorbed by the oceans, leading to an increase in the amount of heat stored by the oceans. The increase of temperature of the oceans goes rather slow, compared to the atmosphere. However, the ocean heat uptake has doubled since 1993 and oceans have absorbed over 90% of the extra heat of the Earth since 1955.[12] The temperature in the ocean, up to approximately 700 meters deep into the ocean, has been rising almost all over the globe.[11] The increased warming in the upper ocean reduces the density of the upper ~500 m of water, while deeper water does not experience as much warming and as great a decrease in density. Thus, the stratification in the upper layers will change more than in the lower layers, and these strengthening vertical density gradients act as barriers limiting mixing between the upper layers and deep-water.
The average global summer stratification of the upper 200 m of the ocean has increased 1.3 ± 0.3% per decade between 1970 and 2018,[13] because warmer summer temperatures heat the upper ocean more than deeper water and cause a slight increase in upper-ocean stratification. However, there is limited evidence that seasonal differences in stratification have grown larger over the years.[8]
Salinity
The salinity is associated with the difference between evaporation and precipitation.[1] Evaporation causes the water to become more saline, and hence denser. Precipitation has the opposite effect, since it decreases the density of the surface water. Hence, it can be stated that salinity plays a more local role in the increase of stratification, even though it is less present compared to the influence of the temperature. For example, salinity plays an important role in the subtropical gyre, North (-East) Pacific, North Atlantic and Arctic regions.[1][14] In the Arctic, the decrease of salinity, and hence density, can be explained by the input of freshwater from melting glaciers and ice sheets. This process and the increase of stratification in the Arctic will continue with the current carbon emissions.[1]
De-oxygenation
A decline in dissolved [math]\displaystyle{ O_2 }[/math], and hence in the [math]\displaystyle{ O_2 }[/math] supply to the ocean interior, is a likely effect of the increase in stratification in the upper ocean.[15] Since [math]\displaystyle{ O_2 }[/math] plays a direct and important role in the cycles of carbon, nitrogen and many other elements such as phosphorus, iron and magnesium, de-oxygenation will have large consequences. The de-oxygenation in subsurface waters is due to the decrease in ocean mixing, which is caused by the increase of stratification in the upper ocean.[1] To illustrate, in the period between 1970 and 1990, approximately 15% of the de-oxygenation can be explained by an increase of temperature and the rest by reduced transport due to stratification.[11] In the period between 1967 and 2000 the decrease in oxygen concentration in the shallow waters, between 0 and 300 meters, was 10 times faster in the coastal ocean compared to the open ocean.[11] This has led to an increase of hypoxic zones, which can lead to a change in behavior of the aquatic flora and fauna. The increase of stratification in the upper ocean during the second half of the 21st century can lead to a decoupling between the surface and the deeper oceans.[14] This decoupling can cause de-oxygenation in the deeper ocean as well, since the decoupling makes it less likely for the oxygen to reach the deeper oceans.
Nevertheless, the change in oxygen concentration can also be influenced by changes in circulation and winds. And even though oxygen has decreased in many areas of the oceans, it can also increase locally, due to a variety of influences on the oxygen. For example, between 1990 and 2000, the oxygen in the thermocline of the Indian Ocean and South Pacific Ocean has increased.[11]
Mixed layer depth
The surface mixed layer is the uppermost layer in the ocean. Due to the turbulence in this layer, generated by for example winds, surface heat fluxes and evaporation, an increase of salinity can occur. The heat stored in the mixed layer can drive phenomena like El Niño. The depth of the mixed layer is associated with physical, chemical and biological systems and is one of the most important quantities in the upper ocean.[16] Throughout the year, the depth of the mixed layer varies. The thickness of the layer increases in wintertime and decreases in the summer. If the mixed layer is really deep, less light can reach the phytoplankton. Phytoplankton have been shown to be important in the global carbon cycle.[17] Furthermore, since phytoplankton are at the bottom of the food chain, a decrease in phytoplankton can have consequences on a very large scale.
An exact relation between an increase in stratification and a change in the mixed layer depth has not yet been determined and remains uncertain. Although some studies suggest that a thinner mixed layer should accompany a more stratified upper ocean,[18][19][20] other work reports seasonal deepening of the mixed layer since 1970.[13] There is literature substantiating the statement that in the years from 1970 to 2018, the stratification in the basis of the mixed layer as well as the depth of the mixed layer have increased. Contradicting this result, other literature states a decrease of the depth of the mixed layer partly as a result of the increase of upper-ocean stratification.[21] It has been found that the mixed layer in the extension of the Kuroshio Current, at the west side of the North Pacific, has decreased with more that 30 meters. This shoaling is caused by weakening of wind and a reduction of seasonal vertical mixing. Furthermore, there exists research stating that heating of the surface of the ocean, and hence an increase in stratification, does not necessarily mean an increase nor decrease in the mixed layer depth.[22] Using the GODAS Data[3] it can be seen that the depth of the mixed layer has increased as well as decreased over time.
Between 1970 and 2018, the summer mixed-layer depth deepened across the globe by 2.9 ± 0.5% (or 3–15 m) per decade, and the Southern Ocean experienced the greatest deepening.[13][22] However, there is limited observational evidence that the mixed layer is globally deepening, and only under strong greenhouse gas emissions scenarios do global mixed-layer depths shoal in the 21st century.[8] Although it is virtually certain that upper ocean stratification will increase through the 21st century, scientists express low confidence in how the mixed-layer depth will evolve.[8]
![]() Annual means and change in annual means of the mixed layer depth of 1980, 2000 and 2020. This plot was generated using the GODAS Data.[3] |
![]() Identification of regions with increase and decrease of annual means of the mixed layer depth of 1980, 2000 and 2020. This plot was generated using the GODAS Data.[3] |
See also
- Earth:Lake stratification – Separation of water in a lake into distinct layers
- Earth:Climate change – Current rise in Earth's average temperature and its effects
References
- ↑ 1.00 1.01 1.02 1.03 1.04 1.05 1.06 1.07 1.08 1.09 1.10 1.11 1.12 1.13 Li, G.; Cheng, L.; Zhu, J.; Trenberth, K.E.; Mann, M.E.; Abraham, J.P. (2020). "Increasing ocean stratification over the past-half century". Nature Climate Change 10 (12): 1116–1123. doi:10.1038/s41558-020-00918-2.
- ↑ Pawlowicz, R. (2013). "Key Physical Variables in the Ocean: Temperature, Salinity and Density". Nature Education Knowledge 4 (4): 13.
- ↑ 3.00 3.01 3.02 3.03 3.04 3.05 3.06 3.07 3.08 3.09 3.10 3.11 "NCEP Global Ocean Data Assimilation System (GODAS) at NOAA ESRL/PSL: NOAA Physical Sciences Laboratory". https://psl.noaa.gov/data/gridded/data.godas.html.
- ↑ 4.0 4.1 Massel, S.R. (2015). Internal gravity waves in the shallow seas. GeoPlanet: Earth and Planetary Sciences. Springer International Publishing. doi:10.1007/978-3-319-18908-6. ISBN 9783319189086. https://link.springer.com/book/10.1007%2F978-3-319-18908-6.
- ↑ Vallis, G.K. (2017). Atmospheric and Oceanic Fluid Dynamics: Fundamentals and Large-Scale Circulation. Cambridge University Press. ISBN 9781107588417.
- ↑ Marsch, R.; van Sebille, E. (n.d.). Ocean Currents: Physical Drivers in a Changing World. Elsevier.
- ↑ Vincent, M.E.; Emanuel, K.A.; Lengaigne, M.; Vialard, J.; Madec, G. (2014). "Influence of upper ocean stratification interannual variability on tropical cyclones". Journal of Advances in Modeling Earth Systems 6 (3): 680–699. doi:10.1002/2014MS000327.
- ↑ 8.0 8.1 8.2 8.3 8.4 Fox-Kemper, B.; Hewitt, H.T.; Xiao, C.; Aðalgeirsdóttir, G.; Drijfhout, S.S.; Edwards, T.L.; Golledge, N.R.; Hemer, M. et al. (2021). Masson-Delmotte, V.; Zhai, P.; Pirani, A. et al.. eds. "Ocean, Cryosphere and Sea Level Change". Climate Change 2021: The Physical Science Basis. Contribution of Working Group I to the Sixth Assessment Report of the Intergovernmental Panel on Climate Change (Cambridge University Press, Cambridge, UK and New York, NY, USA): 1211–1362. doi:10.1017/9781009157896.011.
- ↑ "The Ocean Has Become More Stratified with Global Warming, Study Reveals". 29 September 2020. https://www.ecomagazine.com/news/oceans/the-ocean-has-become-more-stratified-with-global-warming-study-reveals.
- ↑ Snider, L. (28 September 2020). "Climate Change is Creating a Significantly More Stratified Ocean, New Studies Finds". https://news.ucar.edu/132759/climate-change-creating-significantly-more-stratified-ocean-new-study-finds.
- ↑ 11.0 11.1 11.2 11.3 11.4 Rhein, M.; Rintoul, S.R.; Aoki, S.; Campos, E.; Chambers, D.; Feely, R.A.; Gulev, S.; Johnson, G.C. et al. (2013). Climate Change 2013: The Physical Science Basis. Contribution of Working Group I to the Fifth Assessment Report of the Intergovernmental Panel on Climate Change. Cambridge, United Kingdom and New York, USA: Cambridge University Press.
- ↑ 12.0 12.1 "Climate Change Indicators: Ocean Heat". 8 April 2021. https://www.epa.gov/climate-indicators/climate-change-indicators-ocean-heat.
- ↑ 13.0 13.1 13.2 Sallée, J.B.; Pellichero, V.; Akhoudas, C.; Pauthenet, E.; Vignes, L.; Schmidtko, S.; Garabato, A.N.; Sutherland, P. et al. (2021). "Summertime increases in upper-ocean stratification and mixed-layer depth". Nature 591 (7851): 592–598. doi:10.1007/s00382-009-0530-y. PMID 33762764.
- ↑ 14.0 14.1 Capotondi, A.; Alexander, M.A.; Bond, N.A.; Curchitser, E.N.; Scott, J.D. (2012). "Enhanced upper ocean stratification with climate change in the CMIP3 models". Journal of Geophysical Research: Oceans 117 (C4). doi:10.1029/2011JC007409.
- ↑ Keeling, R.F.; Körtzinger, A.; Gruber, N. (2010). "Ocean Deoxygenation in a Warming World". Annual Review of Marine Science 2 (1): 199–229. doi:10.1146/annurev.marine.010908.163855. PMID 21141663.
- ↑ Yeh, S.; Yim, B.Y.; Noh, Y.; Dewitte, B. (2009). "Changes in mixed layer depth under climate change projections in two CGCMs". Climate Dynamics 33 (2–3): 199–213. doi:10.1007/s00382-009-0530-y.
- ↑ Falkowski, P. (2012). "Ocean Science: The power of plankton". Nature 483 (7387): S17–S20. doi:10.1038/483S17a. PMID 22378122.
- ↑ Behrenfeld, Michael J.; O’Malley, Robert T.; Siegel, David A.; McClain, Charles R.; Sarmiento, Jorge L.; Feldman, Gene C.; Milligan, Allen J.; Falkowski, Paul G. et al. (December 2006). "Climate-driven trends in contemporary ocean productivity" (in en). Nature 444 (7120): 752–755. doi:10.1038/nature05317. ISSN 1476-4687. https://www.nature.com/articles/nature05317.
- ↑ Boyce, Daniel G.; Lewis, Marlon R.; Worm, Boris (July 2010). "Global phytoplankton decline over the past century" (in en). Nature 466 (7306): 591–596. doi:10.1038/nature09268. ISSN 1476-4687. https://www.nature.com/articles/nature09268.
- ↑ Polovina, Jeffrey J.; Howell, Evan A.; Abecassis, Melanie (2008-02-14). "Ocean's least productive waters are expanding" (in en). Geophysical Research Letters 35 (3): L03618. doi:10.1029/2007GL031745. ISSN 0094-8276. http://doi.wiley.com/10.1029/2007GL031745.
- ↑ Jang, C.J.; Park, J.; Park, T.; Yoo, S. (2011). "Response of the ocean mixed layer depth to global warming and its impact on primary production: a case for the North Pacific Ocean". ICES Journal of Marine Science 68 (6): 996–1007. doi:10.1093/icesjms/fsr064.
- ↑ 22.0 22.1 Somavilla, R.; Gozález-Pola, C.; Fernández-Diaz, J. (2017). "The warmer the ocean surface, the shallower the mixed layer. How much of this is true?". Journal of Geophysical Research: Oceans 122 (9): 7698–7716. doi:10.1002/2017JC013125. PMID 29201584.
![]() | Original source: https://en.wikipedia.org/wiki/Stratification (water).
Read more |