Biology:Bacterial microcompartment
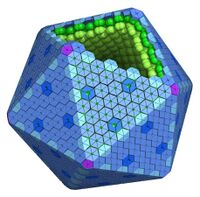
Bacterial microcompartments (BMCs) are organelle-like structures found in bacteria. They consist of a protein shell that encloses enzymes and other proteins. BMCs are typically about 40–200 nanometers in diameter and are made entirely of proteins.[2][3][4][5][6][7][8][9][10][11][12][13] The shell functions like a membrane, as it is selectively permeable.[4][6][8][14][15] Other protein-based compartments found in bacteria and archaea include encapsulin nanocompartments[16] and gas vesicles.[17]
Discovery
The first BMCs were observed in the 1950s in electron micrographs of cyanobacteria,[18] and were later named carboxysomes after their role in carbon fixation was established.[19] Until the 1990s, carboxysomes were thought to be an oddity confined to certain autotrophic bacteria. But then genes coding for proteins homologous to those of the carboxysome shell were identified in the pdu (propanediol utilization)[20] and eut (ethanolamine utilization)[21] operons. Subsequently, transmission electron micrographs of Salmonella cells grown on propanediol[22] or ethanolamine[23] showed the presence of polyhedral bodies similar to carboxysomes. The term metabolosome is used to refer to such catabolic BMCs (in contrast to the autotrophic carboxysome).
Although the carboxysome, propanediol utilizing (PDU), and ethanolamine utilizing (EUT) BMCs encapsulate different enzymes and therefore have different functions, the genes encoding for the shell proteins are very similar. Most of the genes (coding for the shell proteins and the encapsulated enzymes) from experimentally characterized BMCs are located near one another in distinct genetic loci or operons. There are currently over 20,000 bacterial genomes sequenced, and bioinformatics methods can be used to find all BMC shell genes and to look at what other genes are in the vicinity, producing a list of potential BMCs.[2][24][25] In 2014, a comprehensive survey identified 23 different loci encoding up to 10 functionally distinct BMCs across 23 bacterial phyla.[25] In 2021, in an analysis of over 40,000 shell protein sequences, it was shown that at least 45 phyla have members that encode BMCs,[2] and the number of functional types and subtypes has increased to 68.[2] The role of BMCs in the human microbiome is also becoming clear.[26]
Shells
Protein families forming the shell
The BMC shell appears icosahedral[27] or quasi-icosahedral, and is formed by (pseudo)hexameric and pentameric protein subunits.[28] Structures of intact shells have been determined for three functionally distinct: BMC types, carboxysomes,[29] the GRM2 organelles involved in choline catabolism[30] and a metabolosome of unknown function. Collectively, these structures shown that the basic principles of shell assembly are universally conserved across functionally distinct BMCs.[31][28]
The BMC shell protein family
The major constituents of the BMC shell are proteins containing Pfam00936 domain(s). These proteins form oligomers that are hexagonal in shape and form the facets of the shell.
Single-domain proteins (BMC-H)
The BMC-H proteins, which contain a single copy of the Pfam00936 domain, are the most abundant component of the facets of the shell.[28] The crystal structures of a number of these proteins have been determined, showing that they assemble into cyclical hexamers, typically with a small pore in the center.[4] This opening is proposed to be involved in the selective transport of the small metabolites across the shell. Most BMCs contain multiple distinct types of BMC-H proteins (paralogs) that tile together to form the facets, likely reflecting the range of metabolites that must enter and exit the shell.[28]
Tandem-domain proteins (BMC-T)
A subset of shell proteins are composed of tandem (fused) copies of the Pfam00936 domain (BMC-T proteins), this evolutionary event has been recreated in the lab by the construction of a synthetic BMC-T protein.[32] Structurally characterized BMC-T proteins form trimers that are pseudohexameric in shape.[33][34][35] Some BMC-T crystal structures show that the trimers can stack in a face-to-face fashion. In such structures, one pore from one trimer is in an “open” conformation, while the other is closed – suggesting that there may be an airlock-like mechanism that modulates the permeability of some BMC shells.[33][36] This gating appears to be coordinated across the surface of the shell.[31] Another subset of BMC-T proteins contain a [4Fe-4S] cluster, and may be involved in electron transport across the BMC shell.[37][38][39][40][41] Metal centers have also been engineered into BMC-T proteins for conducting electrons.[42][43]
The EutN/CcmL family (BMC-P)
Twelve pentagonal units are necessary to cap the vertices of an icosahedral shell. Crystal structures of proteins from the EutN/CcmL family (Pfam03319) have been solved and they typically form pentamers (BMC-P).[44][45][46] The importance of the BMC-P proteins in shell formation seems to vary among the different BMCs. It was shown that they are necessary for the formation of the shell of the PDU BMC as mutants in which the gene for the BMC-P protein was deleted cannot form shells,[47] but not for the alpha-carboxysome: without BMC-P proteins, carboxysomes will still assemble and many are elongated;[48] these mutant carboxysomes appear to be “leaky”.[49]
Evolution of BMCs and relation to viral capsids
While the BMC shell is architecturally similar to many viral capsids, the shell proteins have not been found to have any structural or sequence homology to capsid proteins. Instead, structural and sequence comparisons suggest that both BMC-H (and BMC-T) and BMC-P, most likely, have evolved from bona fide cellular proteins, namely, PII signaling protein and OB-fold domain-containing protein, respectively.[50]
Permeability of the shell
It is well established that enzymes are packaged within the BMC shell and that some degree of metabolite and cofactor sequestration must occur.[6] However, other metabolites and cofactors must also be allowed to cross the shell in order for BMCs to function. For example, in carboxysomes, ribulose-1,5-bisphosphate, bicarbonate, and phosphoglycerate must cross the shell, while carbon dioxide and oxygen diffusion is apparently limited.[51][52] Similarly, for the PDU BMC, the shell must be permeable to propanediol, propanol, propionyl-phosphate, and potentially also vitamin B12, but it is clear that propionaldehyde is somehow sequestered to prevent cell damage.[53] There is some evidence that ATP must also cross some BMC shells.[6]
It has been proposed that the central pore formed in the hexagonal protein tiles of the shell are the conduits through which metabolites diffuse into the shell.[4][54] For example, the pores in the carboxysome shell have an overall positive charge, which has been proposed to attract negatively charged substrates such as bicarbonate.[4][6][15][54] In the PDU microcompartment, mutagenesis experiments have shown that the pore of the PduA shell protein is the route for entry of the propanediol substrate.[55] For larger metabolites, a gating mechanism in some BMC-T proteins is apparent.[33][36][56] In the EUT microcompartment, gating of the large pore in the EutL shell protein is regulated by the presence of the main metabolic substrate, ethanolamine.[57]
The presence of iron-sulfur clusters in some shell proteins, presumably in the central pore, has led to the suggestion that they can serve as a conduit through which electrons can be shuttled across the shell.[37][40][41]
Types
Comprehensive surveys of microbial genome sequence data indicated more than 60 different metabolic functions encapsulated by BMC shells.[25][2] The majority are involved in either carbon fixation (carboxysomes) or aldehyde oxidation (metabolosomes).[25] A webserver, BMC Caller, allows identification of the BMC type based on the protein sequences of the BMC locus components. BMC Caller
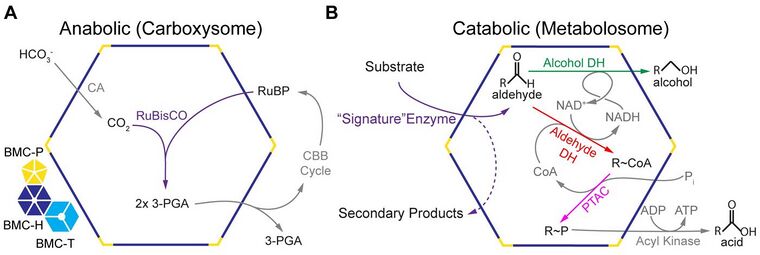
Carboxysomes: carbon fixation
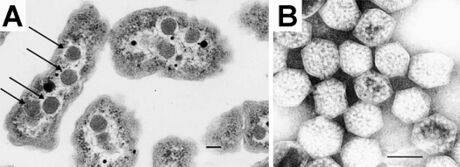
Carboxysomes encapsulate ribulose-1,5-bisphosphate carboxylase/oxygenase (RuBisCO) and carbonic anhydrase in CO
2-fixing bacteria as part of a CO
2 concentrating mechanism.[58] Bicarbonate is pumped into the cytosol and diffuses into the carboxysome, where carbonic anhydrase converts it to carbon dioxide, the substrate of RuBisCO. The carboxysome shell is thought to be only sparingly permeable to carbon dioxide, which results in an effective increase in carbon dioxide concentration around RuBisCO, thus enhancing CO
2 fixation.[52][59] Mutants that lack genes coding for the carboxysome shell display a high CO
2 requiring phenotype due to the loss of the concentration of carbon dioxide, resulting in increased oxygen fixation by RuBisCO. The shells have also been proposed to restrict the diffusion of oxygen,[15][52] thus preventing the oxygenase reaction, reducing wasteful photorespiration.[51]
Metabolosomes: aldehyde oxidation
In addition to the anabolic carboxysomes, several catabolic BMCs have been characterized that participate in the heterotrophic metabolism via short-chain aldehydes; they are collectively termed metabolosomes.[6][23][12]
In 2014 it was proposed that the despite their functional diversity, the majority of metabolosomes share a common encapsulated chemistry driven by three core enzymes: aldehyde dehydrogenase, alcohol dehydrogenase, and phosphotransacylase.[6][25][60][61] Because aldehydes can be toxic to cells[53] and/or volatile,[62] they are thought to be sequestered within the metabolosome. The aldehyde is initially fixed to coenzyme A by a NAD+-dependent aldehyde dehydrogenase, but these two cofactors must be recycled, as they apparently cannot cross the shell.[63][64] These recycling reactions are catalyzed by an alcohol dehydrogenase (NAD+),[63] and a phosphotransacetylase (coenzyme A),[64] resulting in a phosphorylated acyl compound that can readily be a source of substrate-level phosphorylation or enter central metabolism, depending on if the organism is growing aerobically or anaerobically.[53] It seems that most, if not all, metabolosomes utilize these core enzymes. Metabolosomes also encapsulate another enzyme that is specific to the initial substrate of the BMC, that generates the aldehyde; this is the defined signature enzyme of the BMC.[6][25]
PDU BMCs
Some bacteria can use 1,2-propanediol as a carbon source. They use a BMC to encapsulate several enzymes used in this pathway (Sampson and Bobik, 2008). The PDU BMC is typically encoded by a 21 gene locus. These genes are sufficient for assembly of the BMC since they can be transplanted from one type of bacterium to another, resulting in a functional metabolosome in the recipient.[39] This is an example of bioengineering that likewise provides evidence in support of the selfish operon hypothesis.[65] 1,2-propanediol is dehydrated to propionaldehyde by propanediol dehydratase, which requires vitamin B12 as a cofactor.[66] Propionaldehyde causes DNA mutations and as a result is toxic to cells, possibly explaining why this compound is sequestered within a BMC.[53] The end-products of the PDU BMC are propanol and propionyl-phosphate, which is then dephosphorylated to propionate, generating one ATP. Propanol and propionate can be used as substrates for growth.[53]
EUT BMCs
Ethanolamine utilization (EUT) BMCs are encoded in many diverse types of bacteria.[25] Ethanolamine is cleaved to ammonia and acetaldehyde through the action of ethanolamine-ammonia lyase, which also requires vitamin B12 as a cofactor.[67] Acetaldehyde is fairly volatile, and mutants deficient in the BMC shell have been observed to have a growth defect and release excess amounts of acetaldehyde.[62] It has been proposed that sequestration of acetaldehyde in the metabolosome prevents its loss by volatility.[62] The end-products of the EUT BMC are ethanol and acetyl-phosphate. Ethanol is likely a lost carbon source, but acetyl-phosphate can either generate ATP or be recycled to acetyl-CoA and enter the TCA cycle or several biosynthetic pathways.[23]
Bifunctional PDU/EUT BMCs
Some bacteria, especially those in the genus Listeria, encode a single locus in which genes for both PDU and EUT BMCs are present.[25] It is not yet clear whether this is truly a chimeric BMC with a mixture of both sets of proteins, or if two separate BMCs are formed.
Glycyl radical enzyme-containing BMCs (GRM)
Several different BMC loci have been identified that contain glycyl radical enzymes,[24][25][68][69] which obtain the catalytic radical from the cleavage of S-adenosylmethionine.[70] One GRM locus in Clostridium phytofermentans has been shown to be involved in the fermentation of fucose and rhamnose, which are initially degraded to 1,2-propanediol under anaerobic conditions. The glycyl radical enzyme is proposed to dehydrate propanediol to propionaldehyde, which is then processed in a manner identical to the canonical PDU BMC.[71]
Planctomycetes and Verrucomicrobia BMCs (PVM)
Distinct lineages of Planctomycetes and Verrucomicrobia encode a BMC locus. The locus in Planctomyces limnophilus has been shown to be involved in the aerobic degradation of fucose and rhamnose. An aldolase is thought to generate lactaldehyde, which is then processed through the BMC, resulting in 1,2-propanediol and lactyl-phosphate.[60]
Rhodococcus and Mycobacterium BMCs (RMM)
Two types of BMC loci have been observed in members of the Rhodococcus and Mycobacterium genera, although their actual function has not been established.[25] However, based on the characterized function of one of the genes present in the locus and the predicted functions of the other genes, it was proposed that these loci could be involved in the degradation of amino-2-propanol. The aldehyde generated in this predicted pathway would be the extremely toxic compound methylglyoxal; its sequestration within the BMC could protect the cell.[25]
BMCs of unknown function (BUF)
One type of BMC locus does not contain RuBisCO or any of the core metabolosome enzymes, and has been proposed to facilitate a third category of biochemical transformations (i.e. neither carbon fixation nor aldehyde oxidation).[25] The presence of genes predicted to code for amidohydrolases and deaminases could indicate that this BMC is involved in the metabolism of nitrogenous compounds.[25]
Assembly
Carboxysomes
The assembly pathway for beta-carboxysomes has been identified, and begins with the protein CcmM nucleating RuBisCO.[72] CcmM has two domains: an N-terminal gamma-carbonic anhydrase domain followed by a domain consisting of three to five repeats of RuBisCO small-subunit-like sequences.[73] The C-terminal domain aggregates RuBisCO, likely by substituting for the actual RuBisCO small subunits in the L8-S8 holoenzyme, effectively cross-linking the RuBisCO in the cell into one large aggregate, termed the procarboxysome.[72] The N-terminal domain of CcmM physically interacts with the N-terminal domain of the CcmN protein, which, in turn, recruits the hexagonal shell protein subunits via an encapsulation peptide on its C-terminus.[74] Carboxysomes are then spatially aligned in the cyanobacterial cell via interaction with the bacterial cytoskeleton, ensuring their equal distribution into daughter cells.[75]
Alpha-carboxysome assembly may be different than that of beta-carboxysomes,[76] as they have no proteins homologous to CcmN or CcmM and no encapsulation peptides. Empty carboxysomes have been observed in electron micrographs.[77] Some micrographs indicate that their assembly occurs as a simultaneous coalescence of enzymes and shell proteins as opposed to the seemingly stepwise fashion observed for beta-carboxysomes. The formation of simple alpha-carboxysomes in heterologous systems has been shown to require just Rubisco large and small subunits, the internal anchoring protein CsoS2 and the major shell protein CsoS1A.[78]
Phylogenetic analysis of the shell proteins of both types of carboxysomes indicates they independently evolved, each from metabolosome ancestors.[28]
Metabolosomes
Metabolosome assembly is likely similar to that of the beta-carboxysome,[6][72] via an initial aggregation of the proteins to be encapsulated. The core proteins of many metabolosomes aggregate when expressed alone.[79][80][81][82] Moreover, many encapsulated proteins contain terminal extensions that are strikingly similar to the C-terminal peptide of CcmN that recruits shell proteins.[74][83] These encapsulation peptides are short (about 18 residues) and are predicted to form amphipathic alpha-helices.[74] Some of these helices have been shown to mediate the encapsulation of native enzymes into BMCs, as well as heterologous proteins (such as GFP).[74][84][85][86][87]
Regulation (genetic)
With the exception of cyanobacterial carboxysomes, in all tested cases, BMCs are encoded in operons that are expressed only in the presence of their substrate. Genetic loci for the majority of functionally distinct BMC types encode regulator proteins that can provide information about BMC function.[88]
PDU BMCs in Salmonella enterica are induced by the presence of propanediol or glycerol under anaerobic conditions, and only propanediol under aerobic conditions.[89] This induction is mediated by the global regulator proteins Crp and ArcA (sensing cyclic AMP and anaerobic conditions respectively),[90] and the regulatory protein PocR, which is the transcriptional activator for both the pdu and the cob loci (the operon necessary for the synthesis of vitamin B12, a required cofactor for propanediol dehydratase).[89]
EUT BMCs in Salmonella enterica are induced via the regulatory protein EutR by the simultaneous presence of ethanolamine and vitamin B12, which can happen under aerobic or anaerobic conditions. Salmonella enterica can only produce endogenous vitamin B12 under anaerobic conditions, although it can import cyanobalamin and convert it to vitamin B12 under either aerobic or anaerobic conditions.[91]
PVM BMCs in Planctomyces limnophilus are induced by the presence of fucose or rhamnose under aerobic conditions, but not by glucose.[60] Similar results were obtained for the GRM BMC from Clostridium phytofermentans, for which both sugars induce the genes coding for the BMC as well as the ones coding for fucose and rhamnose dissimilatory enzymes.[71]
In addition to characterized regulatory systems, bioinformatics surveys have indicated that there are potentially many other regulatory mechanisms, even within a functional type of BMC (e.g. PDU), including two-component regulatory systems.[25]
Relevance to global and human health
Carboxysomes are present in all cyanobacteria and many other photo- and chemoautotrophic bacteria. Cyanobacteria are globally significant drivers of carbon fixation, and since they require carboxysomes to do so in current atmospheric conditions, the carboxysome is a major component of global carbon dioxide fixation.
Several types of BMCs have been implicated in virulence of pathogens, such as Salmonella enterica and Listeria monocytogenes. BMC genes tend to be upregulated under virulence conditions, and mutating them leads to a virulence defect as judged by competition experiments.[92][93][94][95][96]
Biotechnological applications
Several features of BMCs make them appealing for biotechnological applications. Because carboxysomes increase the efficiency of carbon fixation, much research effort has gone into introducing carboxysomes and required bicarbonate transporters into plant chloroplasts in order to engineer a chloroplastic CO
2 concentrating mechanism[97][98] with some success.[78] Carboxysomes also provide an example of how knowledge of a BMC assembly pathway enables simplification and reduction in the number of necessary gene products for organelle construction.[99] This is an especially important consideration for introducing compartmentalization into difficult to engineer organisms like plants[99][100] in plant synthetic biology.[100][101][99] More generally, because BMC shell proteins self-assemble, empty shells can be formed,[47][102] prompting efforts to engineer them to contain customized cargo. Discovery of the encapsulation peptide on the termini of some BMC-associated proteins[74][84] provides a means to begin to engineer custom BMCs by fusing foreign proteins to this peptide and co-expressing this with shell proteins. For example, by adding this peptide to pyruvate decarboxylase and alcohol dehydrogenase, researchers have engineered an ethanol bioreactor.[103] Strategies for encapsulating proteins into synthetic shells using various adaptor domains[104] and fusions to termini of shell proteins[105] have also been successful. Finally, the pores present in the shell proteins control the permeability of the shell: these can be a target for bioengineering, as they can be modified to allow the crossing of selected substrates and products.[106] The engineering of permeability has even been extended beyond metabolites; shell protein pores have been modified to conduct electrons.[42][43]
In addition to potential for compartmentalizing metabolism in bioengineering,[107] synthetic BMCs have many potential applications as nanotherapeutics.[108] Additional technical advances, such as the ability to construct shells in vitro[109] are rapidly enabling the development of BMCs in biotechnology.
See also
References
- ↑ Sutter, Markus; Greber, Basil; Aussignargues, Clement; Kerfeld, Cheryl A. (2017-06-23). "Assembly principles and structure of a 6.5-MDa bacterial microcompartment shell" (in EN). Science 356 (6344): 1293–1297. doi:10.1126/science.aan3289. PMID 28642439. Bibcode: 2017Sci...356.1293S.
- ↑ 2.0 2.1 2.2 2.3 2.4 Sutter, Markus; Melnicki, Matthew R.; Schulz, Frederik; Woyke, Tanja; Kerfeld, Cheryl A. (December 2021). "A catalog of the diversity and ubiquity of bacterial microcompartments" (in en). Nature Communications 12 (1): 3809. doi:10.1038/s41467-021-24126-4. ISSN 2041-1723. PMID 34155212. Bibcode: 2021NatCo..12.3809S.
- ↑ Cheng, Shouqiang; Liu, Yu; Crowley, Christopher S.; Yeates, Todd O.; Bobik, Thomas A. (2008). "Bacterial microcompartments: their properties and paradoxes". BioEssays 30 (11–12): 1084–1095. doi:10.1002/bies.20830. ISSN 0265-9247. PMID 18937343.
- ↑ 4.0 4.1 4.2 4.3 4.4 "Protein structures forming the shell of primitive bacterial organelles". Science 309 (5736): 936–938. August 2005. doi:10.1126/science.1113397. PMID 16081736. Bibcode: 2005Sci...309..936K.
- ↑ Yeates, Todd O.; Kerfeld, Cheryl A.; Heinhorst, Sabine; Cannon, Gordon C.; Shively, Jessup M. (2008). "Protein-based organelles in bacteria: carboxysomes and related microcompartments". Nature Reviews Microbiology 6 (9): 681–691. doi:10.1038/nrmicro1913. ISSN 1740-1526. PMID 18679172.
- ↑ 6.0 6.1 6.2 6.3 6.4 6.5 6.6 6.7 6.8 Kerfeld, Cheryl A.; Erbilgin, Onur (2015). "Bacterial microcompartments and the modular construction of microbial metabolism". Trends in Microbiology 23 (1): 22–34. doi:10.1016/j.tim.2014.10.003. ISSN 0966-842X. PMID 25455419.
- ↑ "Microcompartments in prokaryotes: carboxysomes and related polyhedra". Applied and Environmental Microbiology 67 (12): 5351–5361. December 2001. doi:10.1128/AEM.67.12.5351-5361.2001. PMID 11722879. Bibcode: 2001ApEnM..67.5351C.
- ↑ 8.0 8.1 Kerfeld, Cheryl A.; Heinhorst, Sabine; Cannon, Gordon C. (2010). "Bacterial Microcompartments". Annual Review of Microbiology 64 (1): 391–408. doi:10.1146/annurev.micro.112408.134211. ISSN 0066-4227. PMID 20825353. PMC 6022854. https://digital.library.unt.edu/ark:/67531/metadc1014927/.
- ↑ Yeates, Todd O.; Crowley, Christopher S.; Tanaka, Shiho (2010). "Bacterial Microcompartment Organelles: Protein Shell Structure and Evolution". Annu. Rev. Biophys. 39 (1): 185–205. doi:10.1146/annurev.biophys.093008.131418. PMID 20192762.
- ↑ Heinhorst, Sabine; Cannon, Gordon C. (2020), Jendrossek, Dieter, ed., "Bacterial Microcompartments" (in en), Bacterial Organelles and Organelle-like Inclusions, Microbiology Monographs (Cham: Springer International Publishing) 34: pp. 125–147, doi:10.1007/978-3-030-60173-7_6, ISBN 978-3-030-60172-0, http://link.springer.com/10.1007/978-3-030-60173-7_6, retrieved 2021-09-17
- ↑ Kennedy, Nolan W; Mills, Carolyn E; Nichols, Taylor M; Abrahamson, Charlotte H; Tullman-Ercek, Danielle (October 2021). "Bacterial microcompartments: tiny organelles with big potential" (in en). Current Opinion in Microbiology 63: 36–42. doi:10.1016/j.mib.2021.05.010. PMID 34126434.
- ↑ 12.0 12.1 Axen, Seth D.; Erbilgin, Onur; Kerfeld, Cheryl A. (2014-10-23). Tanaka, Mark M.. ed. "A Taxonomy of Bacterial Microcompartment Loci Constructed by a Novel Scoring Method" (in en). PLOS Computational Biology 10 (10): e1003898. doi:10.1371/journal.pcbi.1003898. ISSN 1553-7358. PMID 25340524. Bibcode: 2014PLSCB..10E3898A.
- ↑ Mills, C. E.; Waltmann, C.; Archer, A.G.; Kennedy, N.W.; Abrahamson, C.H.; Jackson, A.D.; Roth, E.W.; Shirman, S. et al. (2022). "Vertex protein PduN tunes encapsulated pathway performance by dictating bacterial metabolosome morphology" (in en). Nature Communications 13 (3746): 3746. doi:10.1038/s41467-022-31279-3. PMID 35768404.
- ↑ Yeates, Todd O.; Thompson, Michael C.; Bobik, Thomas A. (2011). "The Protein Shells of Bacterial Microcompartment Organelles". Curr. Opin. Struct. Biol. 21 (2): 223–231. doi:10.1016/j.sbi.2011.01.006. PMID 21315581.
- ↑ 15.0 15.1 15.2 Kinney, James N.; Axen, Seth D.; Kerfeld, Cheryl A. (2011). "Comparative analysis of carboxysome shell proteins". Photosynthesis Research 109 (1–3): 21–32. doi:10.1007/s11120-011-9624-6. ISSN 0166-8595. PMID 21279737.
- ↑ Sutter, Markus; Boehringer, Daniel; Gutmann, Sascha; Günther, Susanne; Prangishvili, David; Loessner, Martin J; Stetter, Karl O; Weber-Ban, Eilika et al. (2008). "Structural basis of enzyme encapsulation into a bacterial nanocompartment". Nature Structural & Molecular Biology 15 (9): 939–947. doi:10.1038/nsmb.1473. ISSN 1545-9993. PMID 19172747.
- ↑ Pfeifer, Felicitas (2012). "Distribution, formation and regulation of gas vesicles". Nature Reviews Microbiology 10 (10): 705–715. doi:10.1038/nrmicro2834. ISSN 1740-1526. PMID 22941504.
- ↑ G. DREWS & W. NIKLOWITZ (1956). "[Cytology of Cyanophycea. II. Centroplasm and granular inclusions of Phormidium uncinatum]". Archiv für Mikrobiologie 24 (2): 147–162. PMID 13327992.
- ↑ "Functional organelles in prokaryotes: polyhedral inclusions (carboxysomes) of Thiobacillus neapolitanus". Science 182 (4112): 584–586. November 1973. doi:10.1126/science.182.4112.584. PMID 4355679. Bibcode: 1973Sci...182..584S.
- ↑ P. Chen, D. I. Andersson & J. R. Roth (September 1994). "The control region of the pdu/cob regulon in Salmonella typhimurium". Journal of Bacteriology 176 (17): 5474–5482. doi:10.1128/jb.176.17.5474-5482.1994. PMID 8071226.
- ↑ I. Stojiljkovic, A. J. Baumler & F. Heffron (March 1995). "Ethanolamine utilization in Salmonella typhimurium: nucleotide sequence, protein expression, and mutational analysis of the cchA cchB eutE eutJ eutG eutH gene cluster". Journal of Bacteriology 177 (5): 1357–1366. doi:10.1128/jb.177.5.1357-1366.1995. PMID 7868611.
- ↑ "The propanediol utilization (pdu) operon of Salmonella enterica serovar Typhimurium LT2 includes genes necessary for formation of polyhedral organelles involved in coenzyme B(12)-dependent 1, 2-propanediol degradation". Journal of Bacteriology 181 (19): 5967–5975. October 1999. doi:10.1128/JB.181.19.5967-5975.1999. PMID 10498708.
- ↑ 23.0 23.1 23.2 Brinsmade, S. R.; Paldon, T.; Escalante-Semerena, J. C. (2005). "Minimal Functions and Physiological Conditions Required for Growth of Salmonella enterica on Ethanolamine in the Absence of the Metabolosome". Journal of Bacteriology 187 (23): 8039–8046. doi:10.1128/JB.187.23.8039-8046.2005. ISSN 0021-9193. PMID 16291677.
- ↑ 24.0 24.1 Jorda, Julien; Lopez, David; Wheatley, Nicole M.; Yeates, Todd O. (2013). "Using comparative genomics to uncover new kinds of protein-based metabolic organelles in bacteria". Protein Science 22 (2): 179–195. doi:10.1002/pro.2196. ISSN 0961-8368. PMID 23188745.
- ↑ 25.00 25.01 25.02 25.03 25.04 25.05 25.06 25.07 25.08 25.09 25.10 25.11 25.12 25.13 25.14 Axen, Seth D.; Erbilgin, Onur; Kerfeld, Cheryl A. (2014). "A Taxonomy of Bacterial Microcompartment Loci Constructed by a Novel Scoring Method". PLOS Computational Biology 10 (10): e1003898. doi:10.1371/journal.pcbi.1003898. ISSN 1553-7358. PMID 25340524. Bibcode: 2014PLSCB..10E3898A.
- ↑ Asija, Kunica; Sutter, Markus; Kerfeld, Cheryl A. (2021-05-13). "A Survey of Bacterial Microcompartment Distribution in the Human Microbiome". Frontiers in Microbiology 12: 669024. doi:10.3389/fmicb.2021.669024. ISSN 1664-302X. PMID 34054778.
- ↑ Vernizzi, G.; Sknepnek, R; Olvera de la Cruz, M. (2011). "Platonic and Archimedean geometries in multi- component elastic membranes" (in en). Proc. Natl. Acad. Sci. USA 108 (11): 4292–4299. doi:10.1073/pnas.1012872108. PMID 21368184.
- ↑ 28.0 28.1 28.2 28.3 28.4 Melnicki, Matthew R.; Sutter, Markus; Kerfeld, Cheryl A. (October 2021). "Evolutionary relationships among shell proteins of carboxysomes and metabolosomes" (in en). Current Opinion in Microbiology 63: 1–9. doi:10.1016/j.mib.2021.05.011. PMID 34098411.
- ↑ Sutter, M.; Laughlin, T.G.; Davies, K.M.; Kerfeld, C.A. (2019-09-25). "Structure of a synthetic beta-carboxysome shell, T=4". Plant Physiology 181 (3): 1050–1058. doi:10.2210/pdb6owg/pdb. PMID 31501298. PMC 6836842. http://dx.doi.org/10.2210/pdb6owg/pdb. Retrieved 2021-09-17.
- ↑ Kalnins, Gints; Cesle, Eva-Emilija; Jansons, Juris; Liepins, Janis; Filimonenko, Anatolij; Tars, Kaspars (December 2020). "Encapsulation mechanisms and structural studies of GRM2 bacterial microcompartment particles" (in en). Nature Communications 11 (1): 388. doi:10.1038/s41467-019-14205-y. ISSN 2041-1723. PMID 31959751. Bibcode: 2020NatCo..11..388K.
- ↑ 31.0 31.1 Greber, Basil J.; Sutter, Markus; Kerfeld, Cheryl A. (May 2019). "The Plasticity of Molecular Interactions Governs Bacterial Microcompartment Shell Assembly". Structure 27 (5): 749–763.e4. doi:10.1016/j.str.2019.01.017. ISSN 0969-2126. PMID 30833088. PMC 6506404. http://dx.doi.org/10.1016/j.str.2019.01.017.
- ↑ Sutter, Markus; McGuire, Sean; Ferlez, Bryan; Kerfeld, Cheryl A. (2019-03-22). "Structural Characterization of a Synthetic Tandem-Domain Bacterial Microcompartment Shell Protein Capable of Forming Icosahedral Shell Assemblies". ACS Synthetic Biology 8 (4): 668–674. doi:10.1021/acssynbio.9b00011. ISSN 2161-5063. PMID 30901520. PMC 6884138. http://dx.doi.org/10.1021/acssynbio.9b00011.
- ↑ 33.0 33.1 33.2 Klein, Michael G.; Zwart, Peter; Bagby, Sarah C.; Cai, Fei; Chisholm, Sallie W.; Heinhorst, Sabine; Cannon, Gordon C.; Kerfeld, Cheryl A. (2009). "Identification and Structural Analysis of a Novel Carboxysome Shell Protein with Implications for Metabolite Transport". Journal of Molecular Biology 392 (2): 319–333. doi:10.1016/j.jmb.2009.03.056. ISSN 0022-2836. PMID 19328811.
- ↑ Sagermann, M.; Ohtaki, A.; Nikolakakis, K. (2009). "Crystal structure of the EutL shell protein of the ethanolamine ammonia lyase microcompartment". Proceedings of the National Academy of Sciences 106 (22): 8883–8887. doi:10.1073/pnas.0902324106. ISSN 0027-8424. PMID 19451619. Bibcode: 2009PNAS..106.8883S.
- ↑ Heldt, Dana; Frank, Stefanie; Seyedarabi, Arefeh; Ladikis, Dimitrios; Parsons, Joshua B.; Warren, Martin J.; Pickersgill, Richard W. (2009). "Structure of a trimeric bacterial microcompartment shell protein, EtuB, associated with ethanol utilization inClostridium kluyveri". Biochemical Journal 423 (2): 199–207. doi:10.1042/BJ20090780. ISSN 0264-6021. PMID 19635047. https://hal.science/hal-00479199/file/PEER_stage2_10.1042%252FBJ20090780.pdf.
- ↑ 36.0 36.1 Cai, F.; Sutter, M.; Cameron, J. C.; Stanley, D. N.; Kinney, J. N.; Kerfeld, C. A. (2013). "The Structure of CcmP, a Tandem Bacterial Microcompartment Domain Protein from the -Carboxysome, Forms a Subcompartment Within a Microcompartment". Journal of Biological Chemistry 288 (22): 16055–16063. doi:10.1074/jbc.M113.456897. ISSN 0021-9258. PMID 23572529.
- ↑ 37.0 37.1 Crowley, Christopher S.; Cascio, Duilio; Sawaya, Michael R.; Kopstein, Jefferey S.; Bobik, Thomas A.; Yeates, Todd O. (2010). "Structural Insight into the Mechanisms of Transport Across the Salmonella Enterica Pdu Microcompartment Shell". Journal of Biological Chemistry 285 (48): 37838–37846. doi:10.1074/jbc.M110.160580. PMID 20870711.
- ↑ Pang, Allan; Warren, Martin J.; Pickersgill, Richard W. (2011). "Structure of PduT, a trimeric bacterial microcompartment protein with a 4Fe–4S cluster-binding site". Acta Crystallographica Section D 67 (2): 91–96. doi:10.1107/S0907444910050201. ISSN 0907-4449. PMID 21245529.
- ↑ 39.0 39.1 Parsons, J. B.; Dinesh, S. D.; Deery, E.; Leech, H. K.; Brindley, A. A.; Heldt, D.; Frank, S.; Smales, C. M. et al. (2008). "Biochemical and Structural Insights into Bacterial Organelle Form and Biogenesis". Journal of Biological Chemistry 283 (21): 14366–14375. doi:10.1074/jbc.M709214200. ISSN 0021-9258. PMID 18332146.
- ↑ 40.0 40.1 Parsons, Joshua B.; Lawrence, Andrew D.; McLean, Kirsty J.; Munro, Andrew W.; Rigby, Stephen E. J.; Warren, Martin J. (2010). "Characterisation of PduS, the pdu Metabolosome Corrin Reductase, and Evidence of Substructural Organisation within the Bacterial Microcompartment". PLOS ONE 5 (11): e14009. doi:10.1371/journal.pone.0014009. ISSN 1932-6203. PMID 21103360. Bibcode: 2010PLoSO...514009P.
- ↑ 41.0 41.1 Thompson, Michael C.; Wheatley, Nicole M.; Jorda, Julien; Sawaya, Michael R.; Gidaniyan, Soheil; Ahmed, Hoda; Yang, Z; McCarty, Crystal et al. (2014). "Identification of a Unique Fe-S Cluster Binding Site in a Glycyl-Radical Type Microcompartment Shell Protein". Journal of Molecular Biology 426 (19): 3287–3304. doi:10.1016/j.jmb.2014.07.018. PMID 25102080.
- ↑ 42.0 42.1 Aussignargues, Clément; Pandelia, Maria-Eirini; Sutter, Markus; Plegaria, Jefferson S.; Zarzycki, Jan; Turmo, Aiko; Huang, Jingcheng; Ducat, Daniel C. et al. (2016-01-11). "Structure and Function of a Bacterial Microcompartment Shell Protein Engineered to Bind a [4Fe-4S Cluster"]. Journal of the American Chemical Society 138 (16): 5262–5270. doi:10.1021/jacs.5b11734. ISSN 0002-7863. PMID 26704697. http://dx.doi.org/10.1021/jacs.5b11734.
- ↑ 43.0 43.1 Plegaria, Jefferson S.; Yates, Matthew D.; Glaven, Sarah M.; Kerfeld, Cheryl A. (2019-12-23). "Redox Characterization of Electrode-Immobilized Bacterial Microcompartment Shell Proteins Engineered To Bind Metal Centers". ACS Applied Bio Materials 3 (1): 685–692. doi:10.1021/acsabm.9b01023. ISSN 2576-6422. PMID 35019413.
- ↑ Tanaka, S.; Kerfeld, C. A.; Sawaya, M. R.; Cai, F.; Heinhorst, S.; Cannon, G. C.; Yeates, T. O. (2008). "Atomic-Level Models of the Bacterial Carboxysome Shell". Science 319 (5866): 1083–1086. doi:10.1126/science.1151458. ISSN 0036-8075. PMID 18292340. Bibcode: 2008Sci...319.1083T.
- ↑ Sutter, Markus; Wilson, Steven C.; Deutsch, Samuel; Kerfeld, Cheryl A. (2013). "Two new high-resolution crystal structures of carboxysome pentamer proteins reveal high structural conservation of CcmL orthologs among distantly related cyanobacterial species". Photosynthesis Research 118 (1–2): 9–16. doi:10.1007/s11120-013-9909-z. ISSN 0166-8595. PMID 23949415. https://escholarship.org/uc/item/930437pp.
- ↑ Wheatley, Nicole M.; Gidaniyan, Soheil D.; Liu, Yuxi; Cascio, Duilio; Yeates, Todd O. (2013). "Bacterial microcompartment shells of diverse functional types possess pentameric vertex proteins". Protein Science 22 (5): 660–665. doi:10.1002/pro.2246. ISSN 0961-8368. PMID 23456886.
- ↑ 47.0 47.1 Parsons, Joshua B.; Frank, Stefanie; Bhella, David; Liang, Mingzhi; Prentice, Michael B.; Mulvihill, Daniel P.; Warren, Martin J. (2010). "Synthesis of Empty Bacterial Microcompartments, Directed Organelle Protein Incorporation, and Evidence of Filament-Associated Organelle Movement". Molecular Cell 38 (2): 305–315. doi:10.1016/j.molcel.2010.04.008. ISSN 1097-2765. PMID 20417607. http://eprints.gla.ac.uk/34075/1/34075.pdf.
- ↑ Yaohua Li, Nolan W. Kennedy, Siyu Li, Carolyn E. Mills, Danielle Tullman-Ercek, Monica Olvera de la Cruz, "Computational and experimental approaches to controlling bacterial microcompartment assembly", ACS Central Science 7, 658–670 (2021); doi.org/10.1021/acscentsci.0c01699
- ↑ Cai, Fei; Menon, Balaraj B.; Cannon, Gordon C.; Curry, Kenneth J.; Shively, Jessup M.; Heinhorst, Sabine (2009). "The Pentameric Vertex Proteins Are Necessary for the Icosahedral Carboxysome Shell to Function as a CO
2 Leakage Barrier". PLOS ONE 4 (10): e7521. doi:10.1371/journal.pone.0007521. ISSN 1932-6203. PMID 19844578. Bibcode: 2009PLoSO...4.7521C. - ↑ Krupovic, M; Koonin, EV (13 November 2017). "Cellular origin of the viral capsid-like bacterial microcompartments.". Biology Direct 12 (1): 25. doi:10.1186/s13062-017-0197-y. PMID 29132422.
- ↑ 51.0 51.1 Marcus, Yehouda; Berry, JosephA.; Pierce, John (1992). "Photosynthesis and photorespiration in a mutant of the cyanobacterium Synechocystis PCC 6803 lacking carboxysomes". Planta 187 (4): 511–6. doi:10.1007/BF00199970. ISSN 0032-0935. PMID 24178146.
- ↑ 52.0 52.1 52.2 Dou, Z.; Heinhorst, S.; Williams, E. B.; Murin, C. D.; Shively, J. M.; Cannon, G. C. (2008). "CO
2 Fixation Kinetics of Halothiobacillus neapolitanus Mutant Carboxysomes Lacking Carbonic Anhydrase Suggest the Shell Acts as a Diffusional Barrier for CO
2". Journal of Biological Chemistry 283 (16): 10377–10384. doi:10.1074/jbc.M709285200. ISSN 0021-9258. PMID 18258595. - ↑ 53.0 53.1 53.2 53.3 53.4 Sampson, E. M.; Bobik, T. A. (2008). "Microcompartments for B12-Dependent 1,2-Propanediol Degradation Provide Protection from DNA and Cellular Damage by a Reactive Metabolic Intermediate". Journal of Bacteriology 190 (8): 2966–2971. doi:10.1128/JB.01925-07. ISSN 0021-9193. PMID 18296526.
- ↑ 54.0 54.1 54.2 "Structural analysis of CsoS1A and the protein shell of the Halothiobacillus neapolitanus carboxysome". PLOS Biology 5 (6): e144. June 2007. doi:10.1371/journal.pbio.0050144. PMID 17518518.
- ↑ Chowdhury, C.; Chun, Sunny; Pang, Allan; Sawaya, Michael R.; Sinha, S.; Yeates, Todd O.; Bobik, Thomas A. (2015). "Selective Molecular Transport Through the Protein Shell of a Bacterial Microcompartment Organelle". Proc. Natl. Acad. Sci. U.S.A. 112 (10): 2990–2995. doi:10.1073/pnas.1423672112. PMID 25713376. Bibcode: 2015PNAS..112.2990C.
- ↑ Tanaka, Shiho; Sawaya, Michael R.; Yeates, Todd O. (2010). "Structure and Mechanisms of a Protein-Based Organelle in Escherichia coli". Science 327 (596): 81–84. doi:10.1126/science.1179513. PMID 20044574. Bibcode: 2010Sci...327...81T.
- ↑ Thompson, Michael C.; Cascio, Duilio; Leibly, David J.; Yeates, Todd O. (2015). "An Allosteric Model for Control of Pore Opening by Substrate Binding in the EutL Microcompartment Shell Protein". Protein Science 24 (6): 956–975. doi:10.1002/pro.2672. PMID 25752492.
- ↑ Murray R. Badger & G. Dean Price (February 2003). "CO2 concentrating mechanisms in cyanobacteria: molecular components, their diversity and evolution". Journal of Experimental Botany 54 (383): 609–622. doi:10.1093/jxb/erg076. PMID 12554704.
- ↑ G. D. Price & M. R. Badger (October 1989). "Expression of Human Carbonic Anhydrase in the Cyanobacterium Synechococcus PCC7942 Creates a High CO(2)-Requiring Phenotype : Evidence for a Central Role for Carboxysomes in the CO(2) Concentrating Mechanism". Plant Physiology 91 (2): 505–513. doi:10.1104/pp.91.2.505. PMID 16667062.
- ↑ 60.0 60.1 60.2 Erbilgin, O.; McDonald, K. L.; Kerfeld, C. A. (2014). "Characterization of a Planctomycetal Organelle: a Novel Bacterial Microcompartment for the Aerobic Degradation of Plant Saccharides". Applied and Environmental Microbiology 80 (7): 2193–2205. doi:10.1128/AEM.03887-13. ISSN 0099-2240. PMID 24487526. Bibcode: 2014ApEnM..80.2193E.
- ↑ Erbilgin, Onur; Sutter, Markus; Kerfeld, Cheryl A. (2016-03-09). "The Structural Basis of Coenzyme A Recycling in a Bacterial Organelle". PLOS Biology 14 (3): e1002399. doi:10.1371/journal.pbio.1002399. ISSN 1545-7885. PMID 26959993.
- ↑ 62.0 62.1 62.2 Joseph T. Penrod & John R. Roth (April 2006). "Conserving a volatile metabolite: a role for carboxysome-like organelles in Salmonella enterica". Journal of Bacteriology 188 (8): 2865–2874. doi:10.1128/JB.188.8.2865-2874.2006. PMID 16585748.
- ↑ 63.0 63.1 Cheng, Shouqiang; Fan, Chenguang; Sinha, Sharmistha; Bobik, Thomas A. (2012). "The PduQ Enzyme Is an Alcohol Dehydrogenase Used to Recycle NAD+ Internally within the Pdu Microcompartment of Salmonella enterica". PLOS ONE 7 (10): e47144. doi:10.1371/journal.pone.0047144. ISSN 1932-6203. PMID 23077559. Bibcode: 2012PLoSO...747144C.
- ↑ 64.0 64.1 Huseby, D. L.; Roth, J. R. (2013). "Evidence that a Metabolic Microcompartment Contains and Recycles Private Cofactor Pools". Journal of Bacteriology 195 (12): 2864–2879. doi:10.1128/JB.02179-12. ISSN 0021-9193. PMID 23585538.
- ↑ J. G. Lawrence & J. R. Roth (August 1996). "Selfish operons: horizontal transfer may drive the evolution of gene clusters". Genetics 143 (4): 1843–1860. doi:10.1093/genetics/143.4.1843. PMID 8844169.
- ↑ R. M. Jeter (May 1990). "Cobalamin-dependent 1,2-propanediol utilization by Salmonella typhimurium". Journal of General Microbiology 136 (5): 887–896. doi:10.1099/00221287-136-5-887. PMID 2166132.
- ↑ D. M. Roof & J. R. Roth (June 1989). "Functions required for vitamin B12-dependent ethanolamine utilization in Salmonella typhimurium". Journal of Bacteriology 171 (6): 3316–3323. doi:10.1128/jb.171.6.3316-3323.1989. PMID 2656649.
- ↑ Ferlez, Bryan; Sutter, Markus; Kerfeld, Cheryl A. (2019-02-26). "Glycyl Radical Enzyme-Associated Microcompartments: Redox-Replete Bacterial Organelles". mBio 10 (1): e02327-18. doi:10.1128/mbio.02327-18. ISSN 2161-2129. PMID 30622187. PMC 6325248. http://dx.doi.org/10.1128/mbio.02327-18.
- ↑ Zarzycki, Jan; Erbilgin, Onur; Kerfeld, Cheryl A. (2015-12-15). "Bioinformatic Characterization of Glycyl Radical Enzyme-Associated Bacterial Microcompartments". Applied and Environmental Microbiology 81 (24): 8315–8329. doi:10.1128/aem.02587-15. ISSN 0099-2240. PMID 26407889. PMC 4644659. Bibcode: 2015ApEnM..81.8315Z. http://dx.doi.org/10.1128/aem.02587-15.
- ↑ Frey, Perry A.; Hegeman, Adrian D.; Ruzicka, Frank J. (2008). "The Radical SAM Superfamily". Critical Reviews in Biochemistry and Molecular Biology 43 (1): 63–88. doi:10.1080/10409230701829169. ISSN 1040-9238. PMID 18307109.
- ↑ 71.0 71.1 Petit, Elsa; LaTouf, W. Greg; Coppi, Maddalena V.; Warnick, Thomas A.; Currie, Devin; Romashko, Igor; Deshpande, Supriya; Haas, Kelly et al. (2013). "Involvement of a Bacterial Microcompartment in the Metabolism of Fucose and Rhamnose by Clostridium phytofermentans". PLOS ONE 8 (1): e54337. doi:10.1371/journal.pone.0054337. ISSN 1932-6203. PMID 23382892. Bibcode: 2013PLoSO...854337P.
- ↑ 72.0 72.1 72.2 Cameron, Jeffrey C.; Wilson, Steven C.; Bernstein, Susan L.; Kerfeld, Cheryl A. (2013). "Biogenesis of a Bacterial Organelle: The Carboxysome Assembly Pathway". Cell 155 (5): 1131–1140. doi:10.1016/j.cell.2013.10.044. ISSN 0092-8674. PMID 24267892.
- ↑ "Analysis of carboxysomes from Synechococcus PCC7942 reveals multiple Rubisco complexes with carboxysomal proteins CcmM and CcaA". The Journal of Biological Chemistry 282 (40): 29323–29335. October 2007. doi:10.1074/jbc.M703896200. PMID 17675289.
- ↑ 74.0 74.1 74.2 74.3 74.4 Kinney, J. N.; Salmeen, A.; Cai, F.; Kerfeld, C. A. (2012). "Elucidating Essential Role of Conserved Carboxysomal Protein CcmN Reveals Common Feature of Bacterial Microcompartment Assembly". Journal of Biological Chemistry 287 (21): 17729–17736. doi:10.1074/jbc.M112.355305. ISSN 0021-9258. PMID 22461622.
- ↑ Savage, D. F.; Afonso, B.; Chen, A. H.; Silver, P. A. (2010). "Spatially Ordered Dynamics of the Bacterial Carbon Fixation Machinery". Science 327 (5970): 1258–1261. doi:10.1126/science.1186090. ISSN 0036-8075. PMID 20203050. Bibcode: 2010Sci...327.1258S.
- ↑ Cai, Fei; Dou, Zhicheng; Bernstein, Susan; Leverenz, Ryan; Williams, Eric; Heinhorst, Sabine; Shively, Jessup; Cannon, Gordon et al. (2015). "Advances in Understanding Carboxysome Assembly in Prochlorococcus and Synechococcus Implicate CsoS2 as a Critical Component". Life 5 (2): 1141–1171. doi:10.3390/life5021141. ISSN 2075-1729. PMID 25826651. Bibcode: 2015Life....5.1141C.
- ↑ Iancu, Cristina V.; Morris, Dylan M.; Dou, Zhicheng; Heinhorst, Sabine; Cannon, Gordon C.; Jensen, Grant J. (2010). "Organization, Structure, and Assembly of α-Carboxysomes Determined by Electron Cryotomography of Intact Cells". Journal of Molecular Biology 396 (1): 105–117. doi:10.1016/j.jmb.2009.11.019. ISSN 0022-2836. PMID 19925807.
- ↑ 78.0 78.1 Long, BM; Hee, WY (2018). "Carboxysome encapsulation of the CO
2-fixing enzyme Rubisco in tobacco chloroplasts.". Nature Communications 9 (1): 3570. doi:10.1038/s41467-018-06044-0. PMID 30177711. Bibcode: 2018NatCo...9.3570L. - ↑ Nicole A. Leal, Gregory D. Havemann & Thomas A. Bobik (November 2003). "PduP is a coenzyme-a-acylating propionaldehyde dehydrogenase associated with the polyhedral bodies involved in B12-dependent 1,2-propanediol degradation by Salmonella enterica serovar Typhimurium LT2". Archives of Microbiology 180 (5): 353–361. doi:10.1007/s00203-003-0601-0. PMID 14504694.
- ↑ Takamasa Tobimatsu, Masahiro Kawata & Tetsuo Toraya (March 2005). "The N-terminal regions of beta and gamma subunits lower the solubility of adenosylcobalamin-dependent diol dehydratase". Bioscience, Biotechnology, and Biochemistry 69 (3): 455–462. doi:10.1271/bbb.69.455. PMID 15784971.
- ↑ "PduL is an evolutionarily distinct phosphotransacylase involved in B12-dependent 1,2-propanediol degradation by Salmonella enterica serovar typhimurium LT2". Journal of Bacteriology 189 (5): 1589–1596. March 2007. doi:10.1128/JB.01151-06. PMID 17158662.
- ↑ Shibata, N.; Tamagaki, H.; Hieda, N.; Akita, K.; Komori, H.; Shomura, Y.; Terawaki, S.-i.; Mori, K. et al. (2010). "Crystal Structures of Ethanolamine Ammonia-lyase Complexed with Coenzyme B12 Analogs and Substrates". Journal of Biological Chemistry 285 (34): 26484–26493. doi:10.1074/jbc.M110.125112. ISSN 0021-9258. PMID 20519496.
- ↑ Aussignargues, Clément; Paasch, Bradley C.; Gonzalez-Esquer, Raul; Erbilgin, Onur; Kerfeld, Cheryl A. (2015). "Bacterial Microcompartment Assembly: The Key Role of Encapsulation Peptides". Communicative & Integrative Biology 8 (3): 00. doi:10.1080/19420889.2015.1039755. ISSN 1942-0889. PMID 26478774.
- ↑ 84.0 84.1 Fan, C.; Cheng, S.; Liu, Y.; Escobar, C. M.; Crowley, C. S.; Jefferson, R. E.; Yeates, T. O.; Bobik, T. A. (2010). "Short N-terminal sequences package proteins into bacterial microcompartments". Proceedings of the National Academy of Sciences 107 (16): 7509–7514. doi:10.1073/pnas.0913199107. ISSN 0027-8424. PMID 20308536. Bibcode: 2010PNAS..107.7509F.
- ↑ Fan, C.; Bobik, T. A. (2011). "The N-Terminal Region of the Medium Subunit (PduD) Packages Adenosylcobalamin-Dependent Diol Dehydratase (PduCDE) into the Pdu Microcompartment". Journal of Bacteriology 193 (20): 5623–5628. doi:10.1128/JB.05661-11. ISSN 0021-9193. PMID 21821773.
- ↑ Choudhary, Swati; Quin, Maureen B.; Sanders, Mark A.; Johnson, Ethan T.; Schmidt-Dannert, Claudia (2012). "Engineered Protein Nano-Compartments for Targeted Enzyme Localization". PLOS ONE 7 (3): e33342. doi:10.1371/journal.pone.0033342. ISSN 1932-6203. PMID 22428024. Bibcode: 2012PLoSO...733342C.
- ↑ Lassila, Jonathan K.; Bernstein, Susan L.; Kinney, James N.; Axen, Seth D.; Kerfeld, Cheryl A. (2014). "Assembly of Robust Bacterial Microcompartment Shells Using Building Blocks from an Organelle of Unknown Function". Journal of Molecular Biology 426 (11): 2217–2228. doi:10.1016/j.jmb.2014.02.025. ISSN 0022-2836. PMID 24631000.
- ↑ Kirst, Henning; Kerfeld, Cheryl A. (2021-06-30). "Clues to the function of bacterial microcompartments from ancillary genes" (in en). Biochemical Society Transactions 49 (3): 1085–1098. doi:10.1042/BST20200632. ISSN 0300-5127. PMID 34196367. PMC 8517908. https://portlandpress.com/biochemsoctrans/article/49/3/1085/229139/Clues-to-the-function-of-bacterial.
- ↑ 89.0 89.1 T. A. Bobik, M. Ailion & J. R. Roth (April 1992). "A single regulatory gene integrates control of vitamin B12 synthesis and propanediol degradation". Journal of Bacteriology 174 (7): 2253–2266. doi:10.1128/jb.174.7.2253-2266.1992. PMID 1312999.
- ↑ M. Ailion, T. A. Bobik & J. R. Roth (November 1993). "Two global regulatory systems (Crp and Arc) control the cobalamin/propanediol regulon of Salmonella typhimurium". Journal of Bacteriology 175 (22): 7200–7208. doi:10.1128/jb.175.22.7200-7208.1993. PMID 8226666.
- ↑ D. E. Sheppard & J. R. Roth (March 1994). "A rationale for autoinduction of a transcriptional activator: ethanolamine ammonia-lyase (EutBC) and the operon activator (EutR) compete for adenosyl-cobalamin in Salmonella typhimurium". Journal of Bacteriology 176 (5): 1287–1296. doi:10.1128/jb.176.5.1287-1296.1994. PMID 8113167.
- ↑ "Identification of Listeria monocytogenes genes contributing to intracellular replication by expression profiling and mutant screening". Journal of Bacteriology 188 (2): 556–568. January 2006. doi:10.1128/JB.188.2.556-568.2006. PMID 16385046.
- ↑ Jochen Klumpp & Thilo M. Fuchs (April 2007). "Identification of novel genes in genomic islands that contribute to Salmonella typhimurium replication in macrophages". Microbiology 153 (Pt 4): 1207–1220. doi:10.1099/mic.0.2006/004747-0. PMID 17379730.
- ↑ "Enterococcus faecalis mutations affecting virulence in the Caenorhabditis elegans model host". Infection and Immunity 75 (5): 2634–2637. May 2007. doi:10.1128/IAI.01372-06. PMID 17307944.
- ↑ Harvey, P. C.; Watson, M.; Hulme, S.; Jones, M. A.; Lovell, M.; Berchieri, A.; Young, J.; Bumstead, N. et al. (2011). "Salmonella enterica Serovar Typhimurium Colonizing the Lumen of the Chicken Intestine Grows Slowly and Upregulates a Unique Set of Virulence and Metabolism Genes". Infection and Immunity 79 (10): 4105–4121. doi:10.1128/IAI.01390-10. ISSN 0019-9567. PMID 21768276.
- ↑ Kendall, M. M.; Gruber, C. C.; Parker, C. T.; Sperandio, V. (2012). "Ethanolamine Controls Expression of Genes Encoding Components Involved in Interkingdom Signaling and Virulence in Enterohemorrhagic Escherichia coli O157:H7". mBio 3 (3): e00050–12–e00050–12. doi:10.1128/mBio.00050-12. ISSN 2150-7511. PMID 22589288.
- ↑ Lin, Myat T.; Occhialini, Alessandro; Andralojc, P. John; Devonshire, Jean; Hines, Kevin M.; Parry, Martin A. J.; Hanson, Maureen R. (2014). "β-Carboxysomal proteins assemble into highly organized structures inNicotianachloroplasts". The Plant Journal 79 (1): 1–12. doi:10.1111/tpj.12536. ISSN 0960-7412. PMID 24810513.
- ↑ Lin, Myat T.; Occhialini, Alessandro; Andralojc, P. John; Parry, Martin A. J.; Hanson, Maureen R. (2014). "A faster Rubisco with potential to increase photosynthesis in crops". Nature 513 (7519): 547–550. doi:10.1038/nature13776. ISSN 0028-0836. PMID 25231869. Bibcode: 2014Natur.513..547L.
- ↑ 99.0 99.1 99.2 Gonzalez‐Esquer, C. Raul; Newnham, Sarah E.; Kerfeld, Cheryl A. (2016-06-20). "Bacterial microcompartments as metabolic modules for plant synthetic biology". The Plant Journal 87 (1): 66–75. doi:10.1111/tpj.13166. ISSN 0960-7412. PMID 26991644.
- ↑ 100.0 100.1 Kerfeld, Cheryl A. (December 2015). "Plug‐and‐play for improving primary productivity". American Journal of Botany 102 (12): 1949–1950. doi:10.3732/ajb.1500409. ISSN 0002-9122. PMID 26656128.
- ↑ Zarzycki, Jan; Axen, Seth D.; Kinney, James N.; Kerfeld, Cheryl A. (2012-10-23). "Cyanobacterial-based approaches to improving photosynthesis in plants". Journal of Experimental Botany 64 (3): 787–798. doi:10.1093/jxb/ers294. ISSN 1460-2431. PMID 23095996.
- ↑ Cai, Fei; Sutter, Markus; Bernstein, Susan L.; Kinney, James N.; Kerfeld, Cheryl A. (2014-08-27). "Engineering Bacterial Microcompartment Shells: Chimeric Shell Proteins and Chimeric Carboxysome Shells". ACS Synthetic Biology 4 (4): 444–453. doi:10.1021/sb500226j. ISSN 2161-5063. PMID 25117559. http://dx.doi.org/10.1021/sb500226j.
- ↑ Lawrence, Andrew D.; Frank, Stefanie; Newnham, Sarah; Lee, Matthew J.; Brown, Ian R.; Xue, Wei-Feng; Rowe, Michelle L.; Mulvihill, Daniel P. et al. (2014). "Solution Structure of a Bacterial Microcompartment Targeting Peptide and Its Application in the Construction of an Ethanol Bioreactor". ACS Synthetic Biology 3 (7): 454–465. doi:10.1021/sb4001118. ISSN 2161-5063. PMID 24933391.
- ↑ Hagen, Andrew; Sutter, Markus; Sloan, Nancy; Kerfeld, Cheryl A. (2018-07-23). "Programmed loading and rapid purification of engineered bacterial microcompartment shells". Nature Communications 9 (1): 2881. doi:10.1038/s41467-018-05162-z. ISSN 2041-1723. PMID 30038362. PMC 6056538. Bibcode: 2018NatCo...9.2881H. http://dx.doi.org/10.1038/s41467-018-05162-z.
- ↑ Ferlez, Bryan; Sutter, Markus; Kerfeld, Cheryl A. (July 2019). "A designed bacterial microcompartment shell with tunable composition and precision cargo loading". Metabolic Engineering 54: 286–291. doi:10.1016/j.ymben.2019.04.011. ISSN 1096-7176. PMID 31075444. PMC 6884132. http://dx.doi.org/10.1016/j.ymben.2019.04.011.
- ↑ Cai, Fei; Sutter, Markus; Bernstein, Susan L.; Kinney, James N.; Kerfeld, Cheryl A. (2015). "Engineering Bacterial Microcompartment Shells: Chimeric Shell Proteins and Chimeric Carboxysome Shells". ACS Synthetic Biology 4 (4): 444–453. doi:10.1021/sb500226j. ISSN 2161-5063. PMID 25117559.
- ↑ Kerfeld, Cheryl A; Sutter, Markus (October 2020). "Engineered bacterial microcompartments: apps for programming metabolism". Current Opinion in Biotechnology 65: 225–232. doi:10.1016/j.copbio.2020.05.001. ISSN 0958-1669. PMID 32554213.
- ↑ Kirst, Henning; Kerfeld, Cheryl A. (2019-10-10). "Bacterial microcompartments: catalysis-enhancing metabolic modules for next generation metabolic and biomedical engineering". BMC Biology 17 (1): 79. doi:10.1186/s12915-019-0691-z. ISSN 1741-7007. PMID 31601225.
- ↑ Hagen, Andrew R.; Plegaria, Jefferson S.; Sloan, Nancy; Ferlez, Bryan; Aussignargues, Clement; Burton, Rodney; Kerfeld, Cheryl A. (2018-10-22). "In Vitro Assembly of Diverse Bacterial Microcompartment Shell Architectures". Nano Letters 18 (11): 7030–7037. doi:10.1021/acs.nanolett.8b02991. ISSN 1530-6984. PMID 30346795. PMC 6309364. Bibcode: 2018NanoL..18.7030H. http://dx.doi.org/10.1021/acs.nanolett.8b02991.
External links
- Mysterious Bacterial Microcompartments Revealed By Biochemists
- Not so simple after all. A renaissance of research into prokaryotic evolution and cell structure
![]() | Original source: https://en.wikipedia.org/wiki/Bacterial microcompartment.
Read more |