Physics:Magneto-optical trap
In atomic, molecular, and optical physics, a magneto-optical trap (MOT) is an apparatus which uses laser cooling and a spatially-varying magnetic field to create a trap which can produce samples of cold, neutral atoms. Temperatures achieved in a MOT can be as low as several microkelvin, depending on the atomic species, which is two or three times below the photon recoil limit. However, for atoms with an unresolved hyperfine structure, such as [[Physics:Isotopes of lithium#Lithium−
7|7
Li]], the temperature achieved in a MOT will be higher than the Doppler cooling limit.
A MOT is formed from the intersection of a weak, quadrupolar, spatially-varying magnetic field and six circularly-polarized, red-detuned, optical molasses beams. As atoms travel away from the field zero at the center of the trap (halfway between the coils), the spatially-varying Zeeman shift brings an atomic transition into resonance which gives rise to a scattering force that pushes the atoms back towards the center of the trap. This is why a MOT traps atoms, and because this force arises from photon scattering in which atoms receive momentum "kicks" in the direction opposite their motion, it also slows the atoms (i.e. cools them), on average, over repeated absorption and spontaneous emission cycles. In this way, a MOT is able to trap and cool atoms with initial velocities of hundreds of meters per second down to tens of centimeters per second (again, depending upon the atomic species).
Although charged particles can be trapped using a Penning trap or a Paul trap using a combination of electric and magnetic fields, those traps are ineffective for neutral atoms.
Theoretical description of a MOT
Two coils in an anti-Helmholtz configuration are used to generate a weak quadrupolar magnetic field; here, we will consider the coils as being separated along the [math]\displaystyle{ z }[/math]-axis. In the proximity of the field zero, located halfway between the two coils along the [math]\displaystyle{ z }[/math]-direction, the field gradient is uniform and the field itself varies linearly with position. For this discussion, consider an atom with ground and excited states with [math]\displaystyle{ J=0 }[/math] and [math]\displaystyle{ J=1 }[/math], respectively, where [math]\displaystyle{ J }[/math] is the magnitude of the total angular momentum vector. Due to the Zeeman effect, these states will each be split into [math]\displaystyle{ 2J+1 }[/math] sublevels with associated values of [math]\displaystyle{ m_J }[/math], denoted by [math]\displaystyle{ |J,m_J\rangle }[/math] (note that the Zeeman shift for the ground state is zero and that it will not be split into sublevels by the field). This results in spatially-dependent energy shifts of the excited-state sublevels, as the Zeeman shift is proportional to the field strength and in this configuration the field strength is linear in position. As a note, the Maxwell equation [math]\displaystyle{ \nabla\cdot\mathbf{B}=0 }[/math] implies that the field gradient is twice as strong along the [math]\displaystyle{ z }[/math]-direction than in the [math]\displaystyle{ x }[/math] and [math]\displaystyle{ y }[/math]-directions, and thus the trapping force along the [math]\displaystyle{ z }[/math]-direction is twice as strong.
In combination with the magnetic field, pairs of counter-propagating circularly-polarized laser beams are sent in along three orthogonal axes, for a total of six MOT beams (there are exceptions to this, but a minimum of five beams is required to make a 3D MOT). The beams are red-detuned from the [math]\displaystyle{ J=0\rightarrow J=1 }[/math] transition by an amount [math]\displaystyle{ \delta }[/math] such that [math]\displaystyle{ \delta\equiv \nu_0- \nu_L \gt 0 }[/math], or equivalently, [math]\displaystyle{ \nu_L=\nu_0-\delta }[/math], where [math]\displaystyle{ \nu_L }[/math] is the frequency of the laser beams and [math]\displaystyle{ \nu_0 }[/math] is the frequency of the transition. The beams must be circularly polarized to ensure that photon absorption can only occur for certain transitions between the ground state [math]\displaystyle{ |0,0\rangle }[/math] and the sublevels of the excited state [math]\displaystyle{ |1,m_J\rangle }[/math], where [math]\displaystyle{ m_J=-1,0,1 }[/math]. In other words, the circularly-polarized beams enforce selection rules on the allowed electric dipole transitions between states.
At the center of the trap, the magnetic field is zero and atoms are "dark" to incident red-detuned photons. That is, at the center of the trap, the Zeeman shift is zero for all states and so the transition frequency [math]\displaystyle{ \nu_0 }[/math] from [math]\displaystyle{ J=0\rightarrow J=1 }[/math] remains unchanged. The detuning of the photons from this frequency means that there will not be an appreciable amount of absorption (and therefore emission) by atoms in the center of the trap, hence the term "dark". Thus, the coldest, slowest moving atoms accumulate in the center of the MOT where they scatter very few photons.
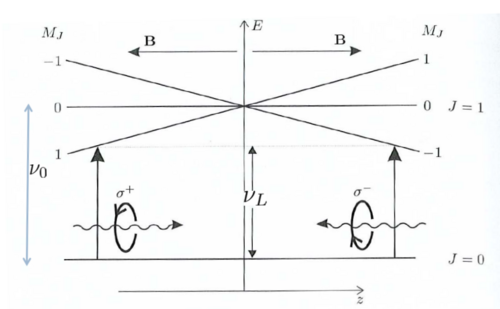
Now consider an atom which is moving in the [math]\displaystyle{ +z }[/math]-direction. The Zeeman effect shifts the energy of the [math]\displaystyle{ |J=1,m_J=-1\rangle }[/math] state lower in energy, decreasing the energy gap between it and the [math]\displaystyle{ |J=0,m_J=0\rangle }[/math] state; that is, the frequency associated with the transition decreases. Red-detuned [math]\displaystyle{ \sigma^- }[/math]photons, which only drive [math]\displaystyle{ \Delta m_J=-1 }[/math] transitions, propagating in the [math]\displaystyle{ -z }[/math]-direction thus become closer to resonance as the atom travels further from the center of the trap, increasing the scattering rate and scattering force. When an atom absorbs a [math]\displaystyle{ \sigma^- }[/math]photon, it is excited to the [math]\displaystyle{ |J=1,m_J=-1\rangle }[/math] state and gets a "kick" of one photon recoil momentum, [math]\displaystyle{ \hbar k }[/math], in the direction opposite to its motion, where [math]\displaystyle{ k=2\pi\nu_0/c }[/math]. The atom, now in an excited state, will then spontaneously emit a photon in a random direction and after many absorption-spontaneous emission events, the atom will have, on average, been "pushed" back towards the field-zero of the trap. This trapping process will also occur for an atom moving in the [math]\displaystyle{ -z }[/math]-direction if [math]\displaystyle{ \sigma^+ }[/math]photons are traveling in the [math]\displaystyle{ +z }[/math]-direction, the only difference being that the excitation will be from [math]\displaystyle{ |J=0,m_J=0\rangle }[/math] to [math]\displaystyle{ |J=1,m_J =+1\rangle }[/math] since the magnetic field is negative for [math]\displaystyle{ z\lt 0 }[/math]. Since the magnetic field gradient near the trap center is uniform, the same phenomenon of trapping and cooling occurs along the [math]\displaystyle{ x }[/math] and [math]\displaystyle{ y }[/math]-directions as well.
Mathematically, the radiation pressure force that atoms experience in a MOT is given by:[2]
[math]\displaystyle{ \mathbf{F}_\mathrm{MOT}=-\alpha\mathbf{v}-\frac{\alpha g \mu_B}{\hbar k}\mathbf{r}\nabla \|\mathbf{B}\|, }[/math]
where [math]\displaystyle{ \alpha }[/math] is the damping coefficient, [math]\displaystyle{ g }[/math] is the Landé g-factor and [math]\displaystyle{ \mu_B }[/math] is the Bohr magneton.
Doppler cooling
Photons have a momentum given by [math]\displaystyle{ \hbar k }[/math] (where [math]\displaystyle{ \hbar }[/math] is the reduced Planck constant and [math]\displaystyle{ k }[/math] the photon wavenumber), which is conserved in all atom-photon interactions. Thus, when an atom absorbs a photon, it is given a momentum kick in the direction of the photon before absorption. By detuning a laser beam to a frequency less than the resonant frequency (also known as red detuning), laser light is only absorbed if the light is frequency up-shifted by the Doppler effect, which occurs whenever the atom is moving towards the laser source. This applies a friction force to the atom whenever it moves towards a laser source.
For cooling to occur along all directions, the atom must see this friction force along all three Cartesian axes; this is most easily achieved by illuminating the atom with three orthogonal laser beams, which are then reflected back along the same direction.
Magnetic trapping
Magnetic trapping is created by adding a spatially varying magnetic quadrupole field to the red detuned optical field needed for laser cooling. This causes a Zeeman shift in the magnetic-sensitive mf levels, which increases with the radial distance from the center of the trap. Because of this, as an atom moves away from the center of the trap, the atomic resonance is shifted closer to the frequency of the laser light, and the atom becomes more likely to get a photon kick towards the center of the trap.
The direction of the kick is given by the polarization of the light, which is either left or right handed circular, giving different interactions with the different mf levels. The correct polarizations are used so that photons moving towards the center of the trap will be on resonance with the correct shifted atomic energy level, always driving the atom towards the center.
Atomic structure necessary for magneto-optical trapping

As a thermal atom at room temperature has many thousands of times the momentum of a single photon, the cooling of an atom must involve many absorption-spontaneous emission cycles, with the atom losing up to ħk of momenta each cycle . Because of this, if an atom is to be laser cooled, it must possess a specific energy level structure known as a closed optical loop, where following an excitation-spontaneous emission event, the atom is always returned to its original state. 85Rubidium, for example, has a closed optical loop between the [math]\displaystyle{ 5S_{1/2}\ F=3 }[/math] state and the [math]\displaystyle{ 5P_{3/2}\ F=4 }[/math] state. Once in the excited state, the atom is forbidden from decaying to any of the [math]\displaystyle{ 5P_{1/2} }[/math] states, which would not conserve parity, and is also forbidden from decaying to the [math]\displaystyle{ 5S_{1/2}\ F=2 }[/math] state, which would require an angular momentum change of −2, which cannot be supplied by a single photon.
Many atoms that do not contain closed optical loops can still be laser cooled, however, by using repump lasers which re-excite the population back into the optical loop after it has decayed to a state outside of the cooling cycle. The magneto-optical trapping of rubidium 85, for example, involves cycling on the closed [math]\displaystyle{ 5S_{1/2}\ F=3\to5P_{3/2}\ F=4 }[/math] transition. On excitation, however, the detuning necessary for cooling gives a small, but non-zero overlap with the [math]\displaystyle{ 5P_{3/2}\ F=3 }[/math] state. If an atom is excited to this state, which occurs roughly every thousand cycles, the atom is then free to decay either the [math]\displaystyle{ F=3 }[/math], light coupled upper hyperfine state, or the [math]\displaystyle{ F=2 }[/math] "dark" lower hyperfine state. If it falls back to the dark state, the atom stops cycling between ground and excited state, and the cooling and trapping of this atom stops. A repump laser which is resonant with the [math]\displaystyle{ 5S_{1/2}\ F=2\to5P_{3/2}\ F=3 }[/math] transition is used to recycle the population back into the optical loop so that cooling can continue.
Apparatus
Laser
All magneto-optical traps require at least one trapping laser plus any necessary repumper lasers (see above). These lasers need stability, rather than high power, requiring no more than the saturation intensity, but a linewidth much less than the Doppler width, usually several megahertz. Because of their low cost, compact size and ease of use, laser diodes are used for many of the standard MOT species while the linewidth and stability of these lasers is controlled using servo systems, which stabilises the lasers to an atomic frequency reference by using, for example, saturated absorption spectroscopy and the Pound-Drever-Hall technique to generate a locking signal.
By employing a 2-dimensional diffraction grating it is possible to generate the configuration of laser beams required for a magneto-optical trap from a single laser beam and thus have a very compact magneto-optical trap.[3]
Vacuum chamber
The MOT cloud is loaded from a background of thermal vapour, or from an atomic beam, usually slowed down to the capture velocity using a Zeeman slower. However, the trapping potential in a magneto-optical trap is small in comparison to thermal energies of atoms and most collisions between trapped atoms and the background gas supply enough energy to the trapped atom to kick it out of the trap. If the background pressure is too high, atoms are kicked out of the trap faster than they can be loaded, and the trap does not form. This means that the MOT cloud only forms in a vacuum chamber with a background pressure of less than 10 micropascals (10−10 bar).
The limits to the magneto-optical trap

The minimum temperature and maximum density of a cloud in a magneto-optical trap is limited by the spontaneously emitted photon in cooling each cycle. While the asymmetry in atom excitation gives cooling and trapping forces, the emission of the spontaneously emitted photon is in a random direction, and therefore contributes to a heating of the atom. Of the two ħk kicks the atom receives in each cooling cycle, the first cools, and the second heats: a simple description of laser cooling which enables us to calculate a point at which these two effects reach equilibrium, and therefore define a lower temperature limit, is known as the Doppler cooling limit.
The density is also limited by the spontaneously emitted photon. As the density of the cloud increases, the chance that the spontaneously emitted photon will leave the cloud without interacting with any further atoms tends to zero. The absorption, by a neighboring atom, of a spontaneously emitted photon gives a 2ħk momentum kick between the emitting and absorbing atom which can be seen as a repulsive force, similar to coulomb repulsion, which limits the maximum density of the cloud.
As of 2022 the method has been demonstrated to work up to triatomic molecules.[4][5]
Application
As a result of low densities and speeds of atoms achieved by optical cooling, the mean free path in a ball of MOT cooled atoms is very long, and atoms may be treated as ballistic. This is useful for quantum information experiments where it is necessary to have long coherence times (the time an atom spends in a defined quantum state). Because of the continuous cycle of absorption and spontaneous emission, which causes decoherence, any quantum manipulation experiments must be performed with the MOT beams turned off. In this case, it is common to stop the expansion of the gases during quantum information experiments by loading the cooled atoms into a dipole trap.
A magneto-optical trap is usually the first step to achieving Bose–Einstein condensation. Atoms are cooled in a MOT down to a few times the recoil limit, and then evaporatively cooled which lowers the temperature and increases the density to the required phase space density.
A MOT of 133Cs was used to make some of the best measurements of CP violation.[citation needed]
MOTs are used in a number of quantum technologies (i.e cold atom gravity gradiometers) and have been deployed on several platforms (i.e UAVs) and in several environments (i.e down boreholes [6]).
See also
- Dipole trap
- Zeeman slower
References
- ↑ {Division of Atomic Physics, Lund University}
- ↑ Foot, C. J. (2005). Atomic physics. Oxford: Oxford University Press. ISBN 978-0-19-152314-4. OCLC 181750270. https://www.worldcat.org/oclc/181750270.
- ↑ Nshii et al.
- ↑ Vilas, Nathaniel B.; Hallas, Christian; Anderegg, Loïc; Robichaud, Paige; Winnicki, Andrew; Mitra, Debayan; Doyle, John M. (6 June 2022). "Magneto-optical trapping and sub-Doppler cooling of a polyatomic molecule" (in en). Nature 606 (7912): 70–74. doi:10.1038/s41586-022-04620-5. ISSN 1476-4687. PMID 35650357. Bibcode: 2022Natur.606...70V. https://www.nature.com/articles/s41586-022-04620-5.
- ↑ L. Miller, Johanna (16 June 2022). "A triatomic molecule is laser cooled and trapped" (in EN). Physics Today 2022 (1): 0616a. doi:10.1063/PT.6.1.20220616a. Bibcode: 2022PhT..2022a.616.. https://physicstoday.scitation.org/do/10.1063/PT.6.1.20220616a/abs/.
- ↑ Vovrosh, Jamie; Wilkinson, Katie; Hedges, Sam; McGovern, Kieran; Hayati, Farzad; Carson, Christopher; Selyem, Adam; Winch, Jonathan et al. (2023). "Magneto-optical trapping in a near-suface borehole". PLOS ONE 18 (7): e0288353. doi:10.1371/journal.pone.0288353. PMID 37432927.
- "The Nobel prize in physics 1997". Nobelprize.org. October 15, 1997. http://nobelprize.org/nobel_prizes/physics/laureates/1997/press.html.
- Raab E. L.; Prentiss M.; Cable A.; Chu S.; Pritchard D.E. (1987). "Trapping of neutral sodium atoms with radiation pressure". Physical Review Letters 59 (23): 2631–2634. doi:10.1103/PhysRevLett.59.2631. PMID 10035608. Bibcode: 1987PhRvL..59.2631R.
- Metcalf, Harold J.; Straten, Peter van der (1999). Laser Cooling and Trapping. Springer-Verlag New York, Inc.. ISBN 978-0-387-98728-6.
- Foot, C.J. (2005). Atomic Physics. Oxford University Press. ISBN 978-0-19-850696-6.
- "Very cold trapped atoms in a vapor cell". Physical Review Letters 65 (13): 1571–1574. 1990-09-24. doi:10.1103/PhysRevLett.65.1571. PMID 10042304. Bibcode: 1990PhRvL..65.1571M. https://zenodo.org/record/1233885.
- Liwag, John Waruel F. Cooling and trapping of 87Rb atoms in a magneto-optical trap using low-power diode lasers, Thesis 621.39767 L767c (1999)
- K B Davis; M O Mewes; M R Andrews; N J van Druten; D S Durfee; D M Kurn; W Ketterle (1997-11-27). "Bose-Einstein Condensation in a Gas of Sodium Atoms". Physical Review Letters 75 (22): 3969–3973. doi:10.1103/PhysRevLett.75.3969. PMID 10059782. Bibcode: 1995PhRvL..75.3969D. https://pure.uva.nl/ws/files/2240246/27876_p3969_1. Retrieved 2019-06-27.
- C. C. Nshii; M. Vangeleyn; J. P. Cotter; P. F. Griffin; E. A. Hinds; C. N. Ironside; P. See; A. G. Sinclair et al. (May 2013). "A surface-patterned chip as a strong source of ultra-cold atoms for quantum technologies". Nature Nanotechnology 8 (5): 321–324. doi:10.1038/nnano.2013.47. PMID 23563845. Bibcode: 2013NatNa...8..321N.
- G. Puentes (July 2020). "Design and Construction of Magnetic Coils for Quantum Magnetism Experiments". Quantum Reports 2 (3): 378–387. doi:10.3390/quantum2030026.
![]() | Original source: https://en.wikipedia.org/wiki/Magneto-optical trap.
Read more |