Biology:Plant communication
Plants are exposed to many stress factors such as disease, temperature changes, herbivory, injury and more. Therefore, in order to respond or be ready for any kind of physiological state, they need to develop some sort of system for their survival in the moment and/or for the future. Plant communication encompasses communication using volatile organic compounds, electrical signaling, and common mycorrhizal networks between plants and a host of other organisms such as soil microbes,[1] other plants[2] (of the same or other species), animals,[3] insects,[4] and fungi.[5] Plants communicate through a host of volatile organic compounds (VOCs) that can be separated into four broad categories, each the product of distinct chemical pathways: fatty acid derivatives, phenylpropanoids/benzenoids, amino acid derivatives, and terpenoids.[6] Due to the physical/chemical constraints most VOCs are of low molecular mass (< 300 Da), are hydrophobic, and have high vapor pressures.[7] The responses of organisms to plant emitted VOCs varies from attracting the predator of a specific herbivore to reduce mechanical damage inflicted on the plant [4] to the induction of chemical defenses of a neighboring plant before it is being attacked.[8] In addition, the host of VOCs emitted varies from plant to plant, where for example, the Venus Fly Trap can emit VOCs to specifically target and attract starved prey.[9] While these VOCs typically lead to increased resistance to herbivory in neighboring plants, there is no clear benefit to the emitting plant in helping nearby plants. As such, whether neighboring plants have evolved the capability to "eavesdrop" or whether there is an unknown tradeoff occurring is subject to much scientific debate.[10] As related to the aspect of meaning-making, the field is also identified as phytosemiotics.[11]
Volatile communication
In Runyon et al. 2006, the researchers demonstrate how the parasitic plant, Cuscuta pentagona (field dodder), uses VOCs to interact with various hosts and determine locations. Dodder seedlings show direct growth toward tomato plants (Lycopersicon esculentum) and, specifically, tomato plant volatile organic compounds. This was tested by growing a dodder weed seedling in a contained environment, connected to two different chambers. One chamber contained tomato VOCs while the other had artificial tomato plants. After 4 days of growth, the dodder weed seedling showed a significant growth towards the direction of the chamber with tomato VOC's. Their experiments also showed that the dodder weed seedlings could distinguish between wheat (Triticum aestivum) VOCs and tomato plant volatiles. As when one chamber was filled with each of the two different VOCs, dodder weeds grew towards tomato plants as one of the wheat VOC's is repellent. These findings show evidence that volatile organic compounds determine ecological interactions between plant species and show statistical significance that the dodder weed can distinguish between different plant species by sensing their VOCs.[12]
Tomato plant to plant communication is further examined in Zebelo et al. 2012, which studies tomato plant response to herbivory. Upon herbivory by Spodoptera littoralis, tomato plants emit VOCs that are released into the atmosphere and induce responses in neighboring tomato plants. When the herbivory-induced VOCs bind to receptors on other nearby tomato plants, responses occur within seconds. The neighboring plants experience a rapid depolarization in cell potential and increase in cytosolic calcium. Plant receptors are most commonly found on plasma membranes as well as within the cytosol, endoplasmic reticulum, nucleus, and other cellular compartments. VOCs that bind to plant receptors often induce signal amplification by action of secondary messengers including calcium influx as seen in response to neighboring herbivory. These emitted volatiles were measured by GC-MS and the most notable were 2-hexenal and 3-hexenal acetate. It was found that depolarization increased with increasing green leaf volatile concentrations. These results indicate that tomato plants communicate with one another via airborne volatile cues, and when these VOC's are perceived by receptor plants, responses such as depolarization and calcium influx occur within seconds.[13]
Terpenoids
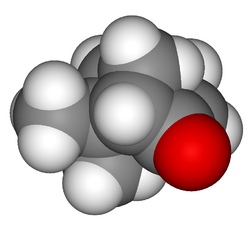
Terpenoids facilitate communication between plants and insects, mammals, fungi, microorganisms, and other plants.[15] Terpenoids may act as both attractants and repellants for various insects. For example, pine shoot beetles (Tomicus piniperda) are attracted to certain monoterpenes ( (+/-)-a-pinene, (+)-3-carene and terpinolene) produced by Scots pines (Pinus sylvestris), while being repelled by others (such as verbenone).[16]
Terpenoids are a large family of biological molecules with over 22,000 compounds.[17] Terpenoids are similar to terpenes in their carbon skeleton but unlike terpenes contain functional groups. The structure of terpenoids is described by the biogenetic isoprene rule which states that terpenoids can be thought of being made of isoprenoid subunits, arranged either regularly or irregularly.[18] The biosynthesis of terpenoids occurs via the methylerythritol phosphate (MEP) and mevalonic acid(MVA) pathways[6] both of which include isopentenyl diphosphate (IPP) and dimethylallyl diphosphate (DMAPP) as key components.[19] The MEP pathway produces hemiterpenes, monoterpenes, diterpenes, and volatile carotenoid derivatives while the MVA pathway produces sesquiterpenes.[6]
Electrical signaling
Many researchers have shown that plants have the ability to use electrical signaling to communicate from leaves to stem to roots. Starting in the late 1800s scientists, such as Charles Darwin, examined ferns and Venus fly traps because they showed excitation patterns similar to animal nerves.[20] However, the mechanisms behind this electrical signaling are not well known and are a current topic of ongoing research.[21] A plant may produce electrical signaling in response to wounding, temperature extremes, high salt conditions, drought conditions, and other various stimuli.[21][22]
There are two types of electrical signals that a plant uses. The first is the action potential and the second is the variation potential.
Similar to action potentials in animals, action potentials in plants are characterized as “all or nothing.”[23] This is the understood mechanism for how plant action potentials are initiated:[24][25][23][26][27][28][29]
- A stimulus transitorily and reversibly activates calcium ion channels
- A short burst of calcium ions into the cell through the open calcium channels
- Calcium ions reversibly inactivate H+-ATPase activity
- Depolarization (due to calcium ion influx) activates voltage gated chloride channels causing chloride ions to leave the cell and cause further depolarization
- Calcium-ATPases decreases intracellular calcium concentration by pumping calcium ions to the outside of the cell (this allows for the H+-ATPase to be reactivated and repolarization to be initiated)
- Repolarization occurs when the activated H+-ATPase pumps H+ out of the cell and the open K+ channels allow for the flow of K+ to the outside of the cell
Plant resting membrane potentials range from -80 to -200 mV.[25][24] High H+-ATPase activity corresponds with hyperpolarization (up to -200mV), making it harder to depolarize and fire an action potential.[24][23][26][30] This is why it is essential for calcium ions to inactivate H+-ATPase activity so that depolarization can be reached.[23][26] When the voltage gated chloride channels are activated and full depolarization occurs, calcium ions are pumped out of the cell (via a calcium-ATPase) after so that H+-ATPase activity resumes so that the cell can repolarize.[23][26]
Calcium's interaction with the H+-ATPase is through a kinase.[26] Therefore, calcium's influx causes the activation of a kinase that phosphorylates and deactivates the H+-ATPase so that the cell can depolarize.[26] It is unclear whether all of the heightened calcium ion intracellular concentration is solely due to calcium channel activation. It is possible that the transitory activation of calcium channels causes an influx of calcium ions into the cell which activates intracellular stores of calcium ions to be released and subsequently causes depolarization (through the inactivation of H+-ATPase and activation of voltage gated chloride channels).[26][27][28][29]
Variation potentials have proven hard to study and their mechanism is less well known than action potentials.[31] Variation potentials are slower than action potentials, are not considered “all or nothing,” and they themselves can trigger several action potentials.[25][31][30][32] The current understanding is that upon wounding or other stressful events, a plant's turgor pressure changes which releases a hydraulic wave throughout the plant that is transmitted through the xylem.[25][33] This hydraulic wave may activate pressure gated channels due to the sudden change in pressure.[34] Their ionic mechanism is very different from action potentials and is thought to involve the inactivation of the P-type H+-ATPase.[25][35]
Long distance electrical signaling in plants is characterized by electrical signaling that occurs over distances greater than the span of a single cell.[36] In 1873, Sir John Burdon-Sanderson described action potentials and their long-distance propagation throughout plants.[32] Action potentials in plants are carried out through a plants vascular network (particularly the phloem),[37] a network of tissues that connects all of the various plant organs, transporting signaling molecules throughout the plant.[36] Increasing the frequency of action potentials causes the phloem to become increasingly cross linked.[38] In the phloem, the propagation of action potentials is dictated by the fluxes of chloride, potassium, and calcium ions, but the exact mechanism for propagation is not well understood.[39] Alternatively, the transport of action potentials over short, local distances is distributed throughout the plant via plasmodesmatal connections between cells.[37]
When a plant responds to stimuli, sometimes the response time is nearly instantaneous which is much faster than chemical signals are able to travel. Current research suggests that electrical signaling may be responsible.[40][41][42][43] In particular, the response of a plant to a wound is triphasic.[41] Phase 1 is an immediate great increase in expression of target genes.[41] Phase 2 is a period of dormancy.[41] Phase 3 is a weakened and delayed upregulation of the same target genes as phase 1.[41] In phase 1, the speed of upregulation is nearly instantaneous which has led researchers to theorize that the initial response from a plant is through action potentials and variation potentials as opposed to chemical or hormonal signaling which is most likely responsible for the phase 3 response.[41][42][43]
Upon stressful events, there is variation in a plant's response. That is to say, it is not always the case that a plant responds with an action potential or variation potential.[41] However, when a plant does generate either an action potential or variation potential, one of the direct effects can be an upregulation of a certain gene's expression.[42] In particular, protease inhibitors and calmodulin exhibit rapid upregulated gene expression.[42] Additionally, ethylene has shown quick upregulation in the fruit of a plant as well as jasmonate in neighboring leaves to a wound.[44][45] Aside from gene expression, action potentials and variation potentials also can result in stomatal and leaf movement.[46][47]
In summary, electric signaling in plants is a powerful tool of communication and controls a plant's response to dangerous stimuli (like herbivory), helping to maintain homeostasis.
Below-ground communication
Chemical Cues
Pisum sativum (garden pea) plants communicate stress cues via their roots to allow neighboring unstressed plants to anticipate an abiotic stressor. Pea plants are commonly grown in temperate regions throughout the world.[48] However, this adaptation allows plants to anticipate abiotic stresses such as drought. In 2011, Falik et al. tested the ability of unstressed pea plants to sense and respond to stress cues by inducing osmotic stress on a neighboring plant.[49] Falik et al. subjected the root of an externally-induced plant to mannitol in order to inflict osmotic stress and drought-like conditions. Five unstressed plants neighbored both sides of this stressed plant. On one side, the unstressed plants shared their root system with their neighbors to allow for root communication. On the other side, the unstressed plants did not share root systems with their neighbors.[49]
Falik et al. found that unstressed plants demonstrated the ability to sense and respond to stress cues emitted from the roots of the osmotically stressed plant. Furthermore, the unstressed plants were able to send additional stress cues to other neighboring unstressed plants in order to relay the signal. A cascade effect of stomatal closure was observed in neighboring unstressed plants that shared their rooting system but was not observed in the unstressed plants that did not share their rooting system.[49] Therefore, neighboring plants demonstrate the ability to sense, integrate, and respond to stress cues transmitted through roots. Although Falik et al. did not identify the chemical responsible for perceiving stress cues, research conducted in 2016 by Delory et al. suggests several possibilities. They found that plant roots synthesize and release a wide array of organic compounds including solutes and volatiles (i.e. terpenes).[50] They cited additional research demonstrating that root-emitted molecules have the potential to induce physiological responses in neighboring plants either directly or indirectly by modifying the soil chemistry.[50] Moreover, Kegge et al. demonstrated that plants perceive the presence of neighbors through changes in water/nutrient availability, root exudates, and soil microorganisms.[51]
Although the underlying mechanism behind stress cues emitted by roots remains largely unknown, Falik et al. suggested that the plant hormone abscisic acid (ABA) may be responsible for integrating the observed phenotypic response (stomatal closure).[49] Further research is needed to identify a well-defined mechanism and the potential adaptive implications for priming neighbors in preparation for forthcoming abiotic stresses; however, a literature review by Robbins et al. published in 2014 characterized the root endodermis as a signaling control center in response to abiotic environmental stresses including drought.[52] They found that the plant hormone ABA regulates the root endodermal response under certain environmental conditions. In 2016 Rowe et al. experimentally validated this claim by showing that ABA regulated root growth under osmotic stress conditions.[53] Additionally, changes in cytosolic calcium concentrations act as signals to close stomata in response to drought stress cues. Therefore, the flux of solutes, volatiles, hormones, and ions are likely involved in the integration of the response to stress cues emitted by roots.
Mycorrhizal networks
Another form of plant communication occurs through their root networks. Through roots, plants can share many different resources including carbon, nitrogen, and other nutrients. This transfer of below ground carbon is examined in Philip et al. 2011. The goals of this paper were to test if carbon transfer was bi-directional, if one species had a net gain in carbon, and if more carbon was transferred through the soil pathway or common mycorrhizal network (CMN). CMNs occur when fungal mycelia link roots of plants together.[54] The researchers followed seedlings of paper birch and Douglas-fir in a greenhouse for 8 months, where hyphal linkages that crossed their roots were either severed or left intact. The experiment measured amounts of labeled carbon exchanged between seedlings. It was discovered that there was indeed a bi-directional sharing of carbon between the two tree species, with the Douglas-fir receiving a slight net gain in carbon. Also, the carbon was transferred through both soil and the CMN pathways, as transfer occurred when the CMN linkages were interrupted, but much more transfer occurred when the CMN's were left unbroken.
This experiment showed that through fungal mycelia linkage of the roots of two plants, plants are able to communicate with one another and transfer nutrients as well as other resources through below ground root networks.[54] Further studies go on to argue that this underground “tree talk” is crucial in the adaptation of forest ecosystems. Plant genotypes have shown that mycorrhizal fungal traits are heritable and play a role in plant behavior. These relationships with fungal networks can be mutualistic, commensal, or even parasitic. It has been shown that plants can rapidly change behavior such as root growth, shoot growth, photosynthetic rate, and defense mechanisms in response to mycorrhizal colonization.[55] Through root systems and common mycorrhizal networks, plants are able to communicate with one another below ground and alter behaviors or even share nutrients depending on different environmental cues.
Acoustic communication
Recent works have shown that plants can respond to airborne sounds at audible frequencies [56] and that they also produce airborne sounds at the ultrasonic range (for example, tomato and tobacco plants when cut with scissors), presumably audible to multiple organisms including bats, mice, moths and other insects.[57]
See also
References
- ↑ Wenke, Katrin; Kai, Marco; Piechulla, Birgit (2010-02-01). "Belowground volatiles facilitate interactions between plant roots and soil organisms". Planta 231 (3): 499–506. doi:10.1007/s00425-009-1076-2. PMID 20012987. Bibcode: 2010Plant.231..499W.
- ↑ Yoneya, Kinuyo; Takabayashi, Junji (2014-01-01). "Plant–plant communication mediated by airborne signals: ecological and plant physiological perspectives". Plant Biotechnology 31 (5): 409–416. doi:10.5511/plantbiotechnology.14.0827a.
- ↑ Leonard, Anne S.; Francis, Jacob S. (2017-04-01). "Plant–animal communication: past, present and future". Evolutionary Ecology 31 (2): 143–151. doi:10.1007/s10682-017-9884-5. Bibcode: 2017EvEco..31..143L.
- ↑ 4.0 4.1 De Moraes, C. M.; Lewis, W. J.; Paré, P. W.; Alborn, H. T.; Tumlinson, J. H. (1998). "Herbivore-infested plants selectively attract parasitoids". Nature 393 (6685): 570–573. doi:10.1038/31219. Bibcode: 1998Natur.393..570D.
- ↑ Bonfante, Paola; Genre, Andrea (2015). "Arbuscular mycorrhizal dialogues: do you speak 'plantish' or 'fungish'?". Trends in Plant Science 20 (3): 150–154. doi:10.1016/j.tplants.2014.12.002. PMID 25583176.
- ↑ 6.0 6.1 6.2 Dudareva, Natalia (April 2013). "Biosynthesis, function and metabolic engineering of plant volatile organic compounds". New Phytologist 198 (1): 16–32. doi:10.1111/nph.12145. PMID 23383981.
- ↑ Rohrbeck, D.; Buss, D.; Effmert, U.; Piechulla, B. (2006-09-01). "Localization of Methyl Benzoate Synthesis and Emission in Stephanotis floribunda and Nicotiana suaveolens Flowers". Plant Biology 8 (5): 615–626. doi:10.1055/s-2006-924076. PMID 16755462. Bibcode: 2006PlBio...8..615R.
- ↑ Baldwin, Jan T.; Schultz, Jack C. (1983). "Rapid Changes in Tree Leaf Chemistry Induced by Damage: Evidence for Communication between Plants". Science 221 (4607): 277–279. doi:10.1126/science.221.4607.277. PMID 17815197. Bibcode: 1983Sci...221..277B.
- ↑ Hedrich, Rainer; Neher, Erwin (March 2018). "Venus Flytrap: How an Excitable, Carnivorous Plant Works" (in en). Trends in Plant Science 23 (3): 220–234. doi:10.1016/j.tplants.2017.12.004. ISSN 1360-1385. PMID 29336976. https://www.cell.com/trends/plant-science/pdf/S1360-1385(17)30280-7.pdf?code=cell-site.
- ↑ Heil, Martin; Karban, Richard (2010-03-01). "Explaining evolution of plant communication by airborne signals". Trends in Ecology & Evolution 25 (3): 137–144. doi:10.1016/j.tree.2009.09.010. ISSN 0169-5347. PMID 19837476.
- ↑ Kull, Kalevi 2000. An introduction to phytosemiotics: Semiotic botany and vegetative sign systems. Sign Systems Studies 28: 326–350.
- ↑ Runyon, Justin B.; Mescher, Mark C.; De Moraes, Consuelo M. (2006-09-29). "Volatile chemical cues guide host location and host selection by parasitic plants". Science 313 (5795): 1964–1967. doi:10.1126/science.1131371. ISSN 1095-9203. PMID 17008532. Bibcode: 2006Sci...313.1964R.
- ↑ Zebelo, Simon A.; Matsui, Kenji; Ozawa, Rika; Maffei, Massimo E. (2012-11-01). "Plasma membrane potential depolarization and cytosolic calcium flux are early events involved in tomato (Solanum lycopersicon) plant-to-plant communication". Plant Science 196: 93–100. doi:10.1016/j.plantsci.2012.08.006. ISSN 0168-9452. PMID 23017903. http://www.sciencedirect.com/science/article/pii/S0168945212001641. Retrieved 2020-10-20.
- ↑ Mafra-Neto, Agenor; de Lame, Frédérique M.; Fettig, Christopher J.; Perring, Thomas M.; Stelinski, Lukasz L.; Stoltman, Lyndsie L.; Mafra, Leandro E. J.; Borges, Rafael et al. (2013). "Manipulation of Insect Behavior with Specialized Pheromone and Lure Application Technology (SPLAT®)". in John Beck. Natural Products for Pest Management. 1141. American Chemical Society. pp. 31–58.
- ↑ Llusià, Joan; Estiarte, Marc; Peñuelas, Josep (1996). "Terpenoids and plant communication". Bull. Inst. Cat. Hist. Nat. 64: 125–133.
- ↑ Byers, J. A.; Lanne, B. S.; Löfqvist, J. (1989-05-01). "Host tree unsuitability recognized by pine shoot beetles in flight". Experientia 45 (5): 489–492. doi:10.1007/BF01952042. ISSN 0014-4754.
- ↑ Hill, Ruaraidh; Connolly, J.D. (1991). Dictionary of terpenoids. Chapman & Hall. ISBN 978-0412257704. OCLC 497430488.
- ↑ Ružička, Leopold (1953). "The isoprene rule and the biogenesis of terpenic compounds". Cellular and Molecular Life Sciences 9 (10): 357–367. doi:10.1007/BF02167631. PMID 13116962.
- ↑ McGarvey, Douglas J.; Croteau, Rodney (July 1995). "Terpenoid Metabolism". The Plant Cell 7 (7): 1015–1026. doi:10.1105/tpc.7.7.1015. PMID 7640522.
- ↑ Darwin, Charles (1875). Insectivorous Plants. London.
- ↑ 21.0 21.1 Hedrich, Rainer; Salvador-Recatalà, Vicenta; Dreyer, Ingo (2016-05-01). "Electrical Wiring and Long-Distance Plant Communication" (in en). Trends in Plant Science 21 (5): 376–387. doi:10.1016/j.tplants.2016.01.016. ISSN 1360-1385. PMID 26880317. https://www.sciencedirect.com/science/article/abs/pii/S1360138516000327.
- ↑ Furch, Alexandra C. U.; Zimmermann, Matthias R.; Will, Torsten; Hafke, Jens B.; van Bel, Aart J. E. (2010-08-01). "Remote-controlled stop of phloem mass flow by biphasic occlusion in Cucurbita maxima". Journal of Experimental Botany 61 (13): 3697–3708. doi:10.1093/jxb/erq181. ISSN 0022-0957. PMID 20584788. PMC 2921205. https://doi.org/10.1093/jxb/erq181.
- ↑ 23.0 23.1 23.2 23.3 23.4 Sukhov, Vladimir; Vodeneev, Vladimir (2009-11-17). "A Mathematical Model of Action Potential in Cells of Vascular Plants" (in en). Journal of Membrane Biology 232 (1): 59–67. doi:10.1007/s00232-009-9218-9. ISSN 1432-1424. PMID 19921324. https://doi.org/10.1007/s00232-009-9218-9.
- ↑ 24.0 24.1 24.2 Sukhov, Vladimir; Nerush, Vladimir; Orlova, Lyubov; Vodeneev, Vladimir (2011-12-21). "Simulation of action potential propagation in plants" (in en). Journal of Theoretical Biology 291: 47–55. doi:10.1016/j.jtbi.2011.09.019. ISSN 0022-5193. PMID 21959317. Bibcode: 2011JThBi.291...47S. https://www.sciencedirect.com/science/article/abs/pii/S0022519311004759.
- ↑ 25.0 25.1 25.2 25.3 25.4 Fromm, Jörg; Lautner, Silke (2007). "Electrical signals and their physiological significance in plants" (in en). Plant, Cell & Environment 30 (3): 249–257. doi:10.1111/j.1365-3040.2006.01614.x. ISSN 1365-3040. PMID 17263772.
- ↑ 26.0 26.1 26.2 26.3 26.4 26.5 26.6 Vodeneev, V. A.; Opritov, V. A.; Pyatygin, S. S. (2006-07-01). "Reversible changes of extracellular pH during action potential generation in a higher plant Cucurbita pepo" (in en). Russian Journal of Plant Physiology 53 (4): 481–487. doi:10.1134/S102144370604008X. ISSN 1608-3407. https://doi.org/10.1134/S102144370604008X.
- ↑ 27.0 27.1 Krol, Elzbieta; Dziubinska, Halina; Trebacz, Kazimierz (2003-05-15). "Low-Temperature Induced Transmembrane Potential Changes in the Liverwort Conocephalum conicum". Plant and Cell Physiology 44 (5): 527–533. doi:10.1093/pcp/pcg070. ISSN 0032-0781. PMID 12773639.
- ↑ 28.0 28.1 Wacke, M.; Thiel, G.; Hütt, M.-T. (2003-02-01). "Ca2+ Dynamics during Membrane Excitation of Green Alga Chara: Model Simulations and Experimental Data" (in en). The Journal of Membrane Biology 191 (3): 179–192. doi:10.1007/s00232-002-1054-0. ISSN 1432-1424. PMID 12571752. https://doi.org/10.1007/s00232-002-1054-0.
- ↑ 29.0 29.1 Tucker, E. B.; Boss, W. F. (June 1996). "Mastoparan-Induced Intracellular Ca2+ Fluxes May Regulate Cell-to-Cell Communication in Plants.". Plant Physiology 111 (2): 459–467. doi:10.1104/pp.111.2.459. ISSN 0032-0889. PMID 12226302.
- ↑ 30.0 30.1 Johns, Sarah; Hagihara, Takuma; Toyota, Masatsugu; Gilroy, Simon (2021-04-02). "The fast and the furious: rapid long-range signaling in plants". Plant Physiology 185 (3): 694–706. doi:10.1093/plphys/kiaa098. ISSN 1532-2548. PMID 33793939.
- ↑ 31.0 31.1 Dziubińska, Halina; Trębacz, Kazimierz; Zawadzki, Tadeusz (2001-01-01). "Transmission route for action potentials and variation potentials in Helianthus annuus L." (in en). Journal of Plant Physiology 158 (9): 1167–1172. doi:10.1078/S0176-1617(04)70143-1. ISSN 0176-1617. https://www.sciencedirect.com/science/article/abs/pii/S0176161704701431.
- ↑ 32.0 32.1 Pickard, Barbara G. (1973). "Action Potentials in Higher Plants". Botanical Review 39 (2): 172–201. doi:10.1007/BF02859299. ISSN 0006-8101. Bibcode: 1973BotRv..39..172P. https://www.jstor.org/stable/4353850.
- ↑ Stankovic, B.; Zawadzki, T.; Davies, E. (November 1997). "Characterization of the Variation Potential in Sunflower.". Plant Physiology 115 (3): 1083–1088. doi:10.1104/pp.115.3.1083. ISSN 0032-0889. PMID 12223859.
- ↑ Farmer, Edward E.; Gao, Yong-Qiang; Lenzoni, Gioia; Wolfender, Jean-Luc; Wu, Qian (2020). "Wound- and mechanostimulated electrical signals control hormone responses" (in en). New Phytologist 227 (4): 1037–1050. doi:10.1111/nph.16646. ISSN 1469-8137. PMID 32392391.
- ↑ Stahlberg, Rainer; Cleland, Robert E.; Van Volkenburgh, Elizabeth (2006), Baluška, František; Mancuso, Stefano; Volkmann, Dieter, eds., "Slow Wave Potentials — a Propagating Electrical Signal Unique to Higher Plants" (in en), Communication in Plants: Neuronal Aspects of Plant Life (Berlin, Heidelberg: Springer): pp. 291–308, doi:10.1007/978-3-540-28516-8_20, ISBN 978-3-540-28516-8, https://doi.org/10.1007/978-3-540-28516-8_20, retrieved 2021-06-03
- ↑ 36.0 36.1 Thain, J.F.; Wildon, D.C. (1992). "Electrical signalling in plants". Science Progress (1933- ) 76 (3/4 (301/302)): 553–564. ISSN 0036-8504. https://www.jstor.org/stable/43421317.
- ↑ 37.0 37.1 Volkov, Alexander G.; Shtessel, Yuri B. (2020-01-01). "Underground electrotonic signal transmission between plants". Communicative & Integrative Biology 13 (1): 54–58. doi:10.1080/19420889.2020.1757207. PMID 32395195. PMC 7202782. https://doi.org/10.1080/19420889.2020.1757207.
- ↑ Calvo, Paco; Sahi, Vaidurya Pratap; Trewavas, Anthony (2017). "Are plants sentient?" (in en). Plant, Cell & Environment 40 (11): 2858–2869. doi:10.1111/pce.13065. ISSN 1365-3040. PMID 28875517. https://onlinelibrary.wiley.com/doi/abs/10.1111/pce.13065.
- ↑ Fromm, Joerg; Hajirezaei, Mohammad-Reza; Becker, Verena Katharina; Lautner, Silke (2013). "Electrical signaling along the phloem and its physiological responses in the maize leaf" (in English). Frontiers in Plant Science 4: 239. doi:10.3389/fpls.2013.00239. ISSN 1664-462X. PMID 23847642.
- ↑ Wildon, D. C.; Thain, J. F.; Minchin, P. E. H.; Gubb, I. R.; Reilly, A. J.; Skipper, Y. D.; Doherty, H. M.; O'Donnell, P. J. et al. (November 1992). "Electrical signalling and systemic proteinase inhibitor induction in the wounded plant" (in en). Nature 360 (6399): 62–65. doi:10.1038/360062a0. ISSN 1476-4687. Bibcode: 1992Natur.360...62W. https://www.nature.com/articles/360062a0.
- ↑ 41.0 41.1 41.2 41.3 41.4 41.5 41.6 Vian, Alain; Henry-Vian, Chantal; Davies, Eric (October 1999). "Rapid and Systemic Accumulation of Chloroplast mRNA-Binding Protein Transcripts after Flame Stimulus in Tomato". Plant Physiology 121 (2): 517–524. doi:10.1104/pp.121.2.517. ISSN 0032-0889. PMID 10517843.
- ↑ 42.0 42.1 42.2 42.3 Stanković, Bratislav; Davies, Eric (1997-07-01). "Intercellular communication in plants: electrical stimulation of proteinase inhibitor gene expression in tomato" (in en). Planta 202 (4): 402–406. doi:10.1007/s004250050143. ISSN 1432-2048. Bibcode: 1997Plant.202..402S. https://doi.org/10.1007/s004250050143.
- ↑ 43.0 43.1 Roux, David; Vian, Alain; Girard, Sébastien; Bonnet, Pierre; Paladian, Françoise; Davies, Eric; Ledoigt, Gérard (2006). "Electromagnetic fields (900 MHz) evoke consistent molecular responses in tomato plants" (in en). Physiologia Plantarum 128 (2): 283–288. doi:10.1111/j.1399-3054.2006.00740.x. ISSN 1399-3054. https://onlinelibrary.wiley.com/doi/abs/10.1111/j.1399-3054.2006.00740.x.
- ↑ Inaba, Akitsugu; Manabe, Taro; Tsuji, Hiroyuki; Iwamoto, Tomotada (1995). "Electrical Impedance Analysis of Tissue Properties Associated with Ethylene Induction by Electric Currents in Cucumber (Cucumis sativus L.) Fruit". Plant Physiology 107 (1): 199–205. doi:10.1104/pp.107.1.199. ISSN 0032-0889. PMID 12228354.
- ↑ Salvador-Recatalà, Vicenta; Tjallingii, W. Freddy; Farmer, Edward E. (2014). "Real-time, in vivo intracellular recordings of caterpillar-induced depolarization waves in sieve elements using aphid electrodes" (in en). New Phytologist 203 (2): 674–684. doi:10.1111/nph.12807. ISSN 1469-8137. PMID 24716546.
- ↑ Devireddy, Amith R.; Arbogast, Jimmie; Mittler, Ron (2020). "Coordinated and rapid whole-plant systemic stomatal responses" (in en). New Phytologist 225 (1): 21–25. doi:10.1111/nph.16143. ISSN 1469-8137. PMID 31454419.
- ↑ Kurenda, Andrzej; Nguyen, Chi Tam; Chételat, Aurore; Stolz, Stéphanie; Farmer, Edward E. (2019-12-17). "Insect-damaged Arabidopsis moves like wounded Mimosa pudica" (in en). Proceedings of the National Academy of Sciences 116 (51): 26066–26071. doi:10.1073/pnas.1912386116. ISSN 0027-8424. PMID 31792188. Bibcode: 2019PNAS..11626066K.
- ↑ "PEA Pisum sativum". https://plants.usda.gov/plantguide/pdf/pg_pisa6.pdf.
- ↑ 49.0 49.1 49.2 49.3 Falik, Omer; Mordoch, Yonat; Quansah, Lydia; Fait, Aaron; Novoplansky, Ariel (2011-11-02). Kroymann, Juergen. ed. "Rumor Has It…: Relay Communication of Stress Cues in Plants" (in en). PLOS ONE 6 (11): e23625. doi:10.1371/journal.pone.0023625. ISSN 1932-6203. PMID 22073135. Bibcode: 2011PLoSO...623625F.
- ↑ 50.0 50.1 Delory, Benjamin M.; Delaplace, Pierre; Fauconnier, Marie-Laure; du Jardin, Patrick (May 2016). "Root-emitted volatile organic compounds: can they mediate belowground plant-plant interactions?" (in en). Plant and Soil 402 (1–2): 1–26. doi:10.1007/s11104-016-2823-3. ISSN 0032-079X. Bibcode: 2016PlSoi.402....1D.
- ↑ Kegge, Wouter; Pierik, Ronald (March 2010). "Biogenic volatile organic compounds and plant competition" (in en). Trends in Plant Science 15 (3): 126–132. doi:10.1016/j.tplants.2009.11.007. PMID 20036599.
- ↑ Robbins, N. E.; Trontin, C.; Duan, L.; Dinneny, J. R. (2014-10-01). "Beyond the Barrier: Communication in the Root through the Endodermis" (in en). Plant Physiology 166 (2): 551–559. doi:10.1104/pp.114.244871. ISSN 0032-0889. PMID 25125504.
- ↑ Rowe, James H.; Topping, Jennifer F.; Liu, Junli; Lindsey, Keith (July 2016). "Abscisic acid regulates root growth under osmotic stress conditions via an interacting hormonal network with cytokinin, ethylene and auxin" (in en). New Phytologist 211 (1): 225–239. doi:10.1111/nph.13882. ISSN 0028-646X. PMID 26889752.
- ↑ 54.0 54.1 Philip, L., S. Simard, and M. Jones. 2010. Pathways for below-ground carbon transfer between paper birch and Douglas-fir seedlings. Plant Ecology & Diversity 3:221–233.
- ↑ Gorzelak, M. A., A. K. Asay, B. J. Pickles, and S. W. Simard. 2015. Inter-plant communication through mycorrhizal networks mediates complex adaptive behaviour in plant communities. AoB Plants 7. Oxford Academic.
- ↑ Veits, M., Khait, I., Obolski, U., Zinger, E., Boonman, A., Goldshtein, A., Saban, K., Seltzer, R., Ben-Dor, U., Estlein, P., Kabat, A., Peretz, D., Ratzersdorfer, I., Krylov, S., Chamovitz, D., Sapir, Y., Yovel, Y. and Hadany, L. (2019), Flowers respond to pollinator sound within minutes by increasing nectar sugar concentration. Ecol Lett, 22: 1483-1492. https://doi.org/10.1111/ele.13331
- ↑ Khait I, Lewin-Epstein O, Sharon R, Saban K, Goldstein R, Anikster Y, Zeron Y, Agassy C, Nizan S, Sharabi G, Perelman R, Boonman A, Sade N, Yovel Y, Hadany L. Sounds emitted by plants under stress are airborne and informative. Cell. 2023 Mar 30;186(7):1328-1336.e10. doi: 10.1016/j.cell.2023.03.009. PMID: 37001499.
Further reading
- Peter Wohlleben; Suzanne Simard; Tim Flannery (2016). The Hidden Life of Trees: What They Feel, How They Communicate―Discoveries from A Secret World. Greystone Books. ISBN 978-1771642484.
![]() | Original source: https://en.wikipedia.org/wiki/Plant communication.
Read more |