Physics:Heterojunction solar cell
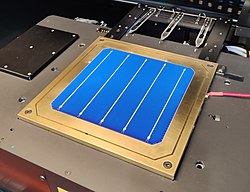

Heterojunction solar cells (HJT), variously known as Silicon heterojunctions (SHJ) or Heterojunction with Intrinsic Thin Layer (HIT),[1] are a family of photovoltaic cell technologies based on a heterojunction formed between semiconductors with dissimilar band gaps. They are a hybrid technology, combining aspects of conventional crystalline solar cells with thin-film solar cells.
Silicon heterojunction-based solar panels are commercially mass-produced for residential and utility markets. As of 2023, Silicon heterojunction architecture has the highest cell efficiency for commercial-sized silicon solar cells.[2] In 2022–2024, SHJ cells are expected to overtake Aluminium Back surface field (Al-BSF) solar cells in market share to become the second-most adopted commercial solar cell technology after PERC/TOPCon (Passivated Emitter Rear Cell/Tunnel Oxide Passivated Contact), increasing to nearly 20% by 2032.[3]
Solar cells operate by absorbing light, exciting the absorber. This creates electron–hole pairs that must be separated into electrons (negative charge carriers) and holes (positive charge carriers) by asymmetry in the solar cell, provided through chemical gradients[4] or electric fields in semiconducting junctions.[5] After splitting, the carriers travel to opposing terminals of the solar cell that have carrier-discriminating properties (known as selective contacts).[6] For solar cells to operate efficiently, surfaces and interfaces require protection from passivation to prevent electrons and holes from being trapped at surface defects, which would otherwise increase the probability of mutual annihilation of the carriers (recombination).
SHJ cells generally consist of an active crystalline silicon absorber substrate which is passivated by a thin layer of hydrogenated intrinsic amorphous silicon (denoted as a-Si:H; the "buffer layer"), and overlayers of appropriately doped amorphous or nanocrystalline silicon selective contacts. The selective contact material and the absorber have different band gaps, forming the carrier-separating heterojunctions that are analogous to the p-n junction of traditional solar cells. The high efficiency of heterojunction solar cells is owed mostly to the excellent passivation qualities of the buffer layers,[7][8][9][10] particularly with respect to separating the highly recombination-active metallic contacts from the absorber.[11] Due to their symmetrical structure, SHJ modules commonly have a bifaciality factor over 90%.[12]
As the thin layers are usually temperature sensitive, heterojunction cells are constrained to a low-temperature manufacturing process.[13][14] This presents challenges for electrode metallisation, as the typical silver paste screen printing method requires firing at up to 800 °C;[15] well above the upper tolerance for most buffer layer materials. As a result, the electrodes are composed of a low curing temperature silver paste, or uncommonly[3] a silver-coated copper paste or electroplated copper.
History
The heterojunction structure, and the ability of amorphous silicon layers to effectively passivate crystalline silicon has been well documented since the 1970s.[9][16][17] Heterojunction solar cells using amorphous and crystalline silicon were developed with a conversion efficiency of more than 12% in 1983.[18] Sanyo Electric Co. (now a subsidiary of Panasonic Group) filed several patents pertaining to heterojunction devices including a-Si and μc-Si intrinsic layers in the early 1990s, trademarked "heterojunction with intrinsic thin-layer" (HIT).[19][20] The inclusion of the intrinsic layer significantly increased efficiency over doped a-Si heterojunction solar cells through reduced density of trapping states, and reduced dark tunnelling leakage currents.[21]
Research and development of SHJ solar cells was suppressed until the expiry of Sanyo-issued patents in 2011, allowing various companies to develop SHJ technology for commercialisation.[22][11] In 2014, HIT cells with conversion efficiencies exceeding 25% were developed by Panasonic, which was then the highest for non-concentrated crystalline silicon cells.[23] This record was broken more recently in 2018 by Kaneka corporation, which produced 26.7% efficient large area interdigitated back contact (IBC) SHJ solar cells,[24] and again in 2022 and 2023 by LONGi with 26.81%[25] and 27.09%[26] efficiency respectively. As of 2023, this is the highest recorded efficiency for non-concentrated crystalline silicon solar cells.[2][27] Heterojunction modules have been fabricated with efficiency up to 23.89%.[28] In 2023, SHJ combined with Perovskite in monolithic tandem cells also recorded the highest non-concentrated Two-junction cell efficiency at 33.9%.[29]
SHJ solar cells are now mass-produced on the gigawatt scale. In 2022, projects planned for the establishment or expansion of SHJ production lines totaled approximately 350 GW/year of additional capacity.[30] Over 24 (mostly Chinese) manufacturers are beginning or augmenting their heterojunction production capacity, such as Huasun, Risen, Jingang (Golden Glass), LONGi, Meyer Burger and many more.[31]
Utility scale projects
In early 2022, a 150 MW heterojunction solar farm was completed by Bulgarian EPC company Inercom near the village of Apriltsi in Pazardzhik Province, Bulgaria—the largest HJT solar farm at the time, according to a press release by module supplier Huasun.[32] In 2023, the same supplier announced a further 1.5 GW supply deal of HJT modules to Inercom.[33]
Advantages
Performance
Efficiency and voltage
SHJ has the highest efficiency amongst crystalline silicon solar cells in both laboratory (world record efficiency)[2][27][29] and commercial production (average efficiency). In 2023, the average efficiency for commercial SHJ cells was 25.0%, compared with 24.9% for n-type TOPCon and 23.3% for p-type PERC.[34] The high efficiency is owed mostly to very high open-circuit voltages—consistently over 700 mV—as a result of excellent surface passivation. Since 2023, SHJ bottom cells in Perovskite tandems also hold the highest non-concentrated Two-junction cell efficiency at 33.9%.[29] Due to their superior surface passivation, heterojunction cells generally have a lower diode saturation current density than other silicon solar cells (such as TOPCon), allowing for very high fill factor and voltage; and hence record high efficiency.[35]
Bifaciality
Bifaciality refers to the ability of a solar cell to accept light from the front or rear surface. The collection of light from the rear surface can significantly improve energy yields in deployed solar arrays.[36] SHJ cells can be manufactured with a conductive ARC on both sides, allowing a bifaciality factor above 90%, compared to ~70% for PERC cells with rear grid.[12] Bifacial solar modules are expected to significantly increase their market share over monofacial modules to 85% by 2032.[3]
Lifespan
By virtue of their high bifaciality, silicon heterojunction modules can exploit more advantages of glass–glass module designs compared to other cell technologies. Glass–glass modules using EPE encapsulant are particularly effective in preventing water ingress, which is a significant cause of performance degradation in PV modules. When used with the appropriate module encapsulant, a glass–glass SHJ module is generally expected to have an operational lifespan of over 30 years; significantly longer than a glass–polymer foil backsheet (the module technology with the highest market share as of 2023). Glass–glass modules are heavier than glass–backsheet modules, however due to improvement in tempered glass technologies and module designs, the glass thickness (and hence weight) is expected to reduce, with the mainstream tending from 3.2 mm towards 2 mm or less in the 2030s.[37][38] As a result, glass–glass modules are expected to become the dominant PV technology in the mid 2020s according to ITRPV (2023).
For example, utility scale 680 W heterojunction modules with a 30-year performance derating of 93% were announced by Enel in 2022.[39]
Temperature coefficient
The temperature coefficient refers to how the output power of a solar module changes with temperature. Typically, solar modules see a reduction in output power and efficiency at elevated temperatures. From lab testing and supplier datasheet surveys, modules fabricated with SHJ cells consistently measure an equal or lower temperature coefficient (i.e. the decrease in efficiency is less severe) compared with Al-BSF, PERC, PERT and hybrid PERT/rear-heterojunction solar cells. This applies to a range of parameters, including open-circuit voltage, maximum power point power, short circuit current and fill factor.[40] The temperature sensitivity of solar cells has been inversely correlated to high open-circuit voltages compared to the absorber band gap potential,[41] as noted by Martin Green in 1982; "As the open-circuit voltage of silicon solar cells continues to improve, one resulting advantage, not widely appreciated, is reduced temperature sensitivity of device performance".[42] Thus the low temperature sensitivity of SHJ cells has been attributed to high [math]\displaystyle{ V_{OC} }[/math] from well passivated contacts.[43]
Manufacturing
Energy consumption
SHJ production lines fundamentally do not use high temperature equipment such as diffusion or metal paste curing furnaces,[22] and on average have a lower power consumption per watt of fabricated cells. According to China PV Industry Development Roadmap, in 2022, the average electricity consumption of n-type Heterojunction cell lines was 47,000 kWh/MW, whereas p-type PERC production lines consumed about 53,000 kWh/MW and for n-type TOPCon, about 56,000 kWh/MW. It is estimated that by 2030, the power consumption of n-type Heterojunction, p-type PERC and n-type TOPCon cell production lines will drop to 34,000 kWh/MW, 35,000 kWh/MW and 42,000 kWh/MW respectively.[34] A 2014 study estimated the energy payback time of a SHJ module to be 1.5 years, compared to 1.8 years for a regular monocrystalline module; this figure was estimated to drop to 0.94 years vs. 1.2 years respectively for a prospective module in 2020 assuming 25% efficiency. Similarly, the life-cycle CO2-equivalent emissions per kWh for 2020 SHJ modules is estimated to be 20 grams vs 25 grams for a regular monocrystalline module.[44]
Silicon consumption
Crystalline silicon wafers used in solar cells typically have a thickness between 130 and 180 μm. The mass of consumed silicon wafer comprises a significant proportion of the cost of the solar module, and as such reducing the wafer thickness has potential to achieve significant cost reduction.[45] Fewer photons are absorbed in thinner silicon. However, as long as surface recombination is effectively suppressed, thinner wafers can maintain—or even improve upon—very high open-circuit voltages.[46] That is, the increase in open-circuit voltage may compensate for losses in short-circuit current. They do so fundamentally,[47][48] as a greater proportion of recombination occurs in the bulk of the substrate if surfaces are well passivated, therefore reducing the thickness reduces the quantity of bulk defects.[49] As SHJ cells have excellent surface passivation, reduction in their wafer thickness is more feasible than with other crystalline silicon solar cell technologies.[22][12] As such, high efficiencies have been reported over a large range of wafer thicknesses, with the minimum on the order of 50 μm.[50] On commercial-grade n-type substrates, the optimum thickness is estimated to be 40–60 μm.[49] This advantage is not seen in technologies with non-passivated contacts or poor surface recombination such as PERC, in which the optimum thickness is greater than 100 μm.[45]
Disadvantages
Cost
Operational expenditure
SHJ modules are estimated to be approximately 3-4 ¢/Wp more expensive than PERC modules (both assuming Chinese manufacturing; sources cite 2018 benchmark). The majority of the increased operational expenditure is due to differences in metallisation technology, which was estimated to be responsible for about 1.8 ¢/Wp of that difference.[51] The cost of PECVD for a-Si and sputtering for TCO layers were also significant contributors to cost increases. Other factors include higher cost of n-type wafers, as well as surface preparation.[52]
Capital expenditure
In 2020, the CapEx cost for SHJ was much higher than PERC. The major cost (up to 50%) of establishing a SHJ production line is attributed to the PECVD equipment. However, SHJ production line CapEx has been trending downward mostly due to the reduction in PECVD tool price, from $USD 125M before 2018 to $USD30–40 M at the end of 2020. As of 2021, the CapEx of SHJ production lines in Europe was still significantly greater than in China.[52] Higher tool throughput also reduces the CapEx cost per gigawatt. In 2019, leading PECVD equipment capacity was below 3000 wafers/hour (manufactured by Meyer Burger, INDEOtec and Archers Suzhou Systems), with newer PECVD tools (such as those manufactured by Maxwell and GS Solar) increasing capacity to 5000–8000 wafers/hour.[53]
Manufacturing
Reliance on n-type silicon
Although high efficiency SHJ cells can be manufactured using a p-type silicon substrate, the low temperature constraint on SHJ production makes the process of gettering (management of contamination defects) impossible and bulk hydrogenation cannot reliably passivate excessive defects. For the same concentration of contaminant transition metal defects, n-type wafers have a higher minority carrier lifetime due to the smaller capture cross section of holes (the minority charge carrier) compared to electrons. Similarly, the capture cross section ratio of electrons to holes is large for surface states (eg. silicon dangling bonds) and therefore well passivated surfaces are easier to achieve on n-type wafers.[11][43] For these reasons, n-type wafers are strongly preferred for manufacturing, as inconvenient steps for improving bulk lifetimes are cut out and the risk of developing light-induced degradation is reduced.[54] However, the cost of n-type wafers is usually cited to be about 8–10% higher than p-type.[54][55]
The higher price of n-type wafers is attributed to the smaller segregation coefficient of phosphorus in silicon whilst growing of doped monocrystalline ingots. This results in a problematic variation in resistivity across the length of the ingot,[56] and thus only about 75% of the volume meets the resistivity tolerance as required by PV manufacturers.[52] Furthermore, n-type ingots grown in crucibles that have been reused many times (rechargeable Czochralski; RCz) are less likely to be acceptable.[52][57]
Surface preparation and texturing
One of the first steps in manufacturing crystalline silicon solar cells includes texturing and cleaning the surface of the silicon wafer substrate. For monocrystalline wafers, this involves an anisotropic wet chemical etch using a mixture of an alkaline solution (usually potassium hydroxide or metal ion-free tetramethylammonium hydroxide) and an organic additive to increase etching anisotropy[58] (traditionally isopropyl alcohol, but now proprietary additives are used). The etch forms the light-trapping pyramidal texture that improves the output current of the finished solar cell. Due to stringent requirements for surface cleanliness for SHJ compared to PERC, the texturing and cleaning process is relatively more complex and consumes more chemicals. Some of these surface treatment steps include RCA cleaning, sulfuric acid/peroxide mixtures to remove organics, removal of metal ions using hydrochloric acid, and nitric acid oxidative cleaning and etch-backs.[59] Recent developments in research has found that oxidative cleaning with ozonated water may help improve process efficiency and reduce waste, with the possibility of completely replacing RCA cleaning whilst maintaining the same surface quality.[59][60]
Silver paste screen printing
The vast majority of solar cells are manufactured with screen-printed paste electrodes. SHJ cells are constrained to a low-temperature process and thus cannot use traditional furnace-fired silver paste for their electrodes, such as what is used in PERC, TOPCon and Al-BSF cells. The low-temperature paste composition compromises several factors in the performance and economics of SHJ, such as high silver consumption and lower grid conductivity.[61][62] Furthermore, the screen printing process of low-temperature silver paste onto SHJ cells also generally has a significantly lower throughput compared to PERC screen printing lines, as manufacturers often use a lower printing and flooding velocity to achieve a high quality grid.[63] Terawatt-scale solar is anticipated to consume a significant fraction of global silver demand unless alternatives are developed.[64] Emerging technologies that may reduce silver consumption for SHJ include silver-coated copper paste, silver nanoparticle ink, and electroplated copper.
Technological maturity
SHJ production lines consist mostly of new equipment. Therefore, SHJ experiences difficulties competing with TOPCon production, as existing PERC production lines can be retrofitted for TOPCon relatively easily. A report by Wood Mackenzie (Dec 2022) predicts that TOPCon will be favoured over SHJ for new module production in the United States in light of the Inflation Reduction Act for this reason, citing a preferable balance between high efficiency and capital expenditure.[65]
Structure

A "front-junction" heterojunction solar cell is composed of a p–i–n–i–n-doped stack of silicon layers; the middle being an n-type crystalline silicon wafer and the others being amorphous thin layers. Then, overlayers of a transparent conducting oxide (TCO) antireflection coating and metal grid are used for light and current collection. Due to the high bifaciality of the SHJ structure, the similar n–i–n–i–p "rear-junction" configuration is also used by manufacturers and may have advantages depending on the process.[66] In particular, rear-junction configurations are preferred in manufacturing as they allow for a greater proportion of lateral electron transport to transpire in the absorber rather than the front TCO. Therefore, the sheet resistance of the front side is lowered and restrictions on TCO process parameters are relaxed, leading to efficiency and cost benefits.[67][68]
Absorber
The substrate, in which electron-hole pairs are formed, is usually n-type monocrystalline silicon doped with phosphorus. In industrial production of high efficiency SHJ solar cells, high quality n-type Czochralski silicon is required because the low-temperature process cannot provide the benefits of gettering and bulk hydrogenation.[15][69] Photons absorbed outside the substrate do not contribute to photocurrent and constitute losses in quantum efficiency.
Buffer and carrier selection
Buffer Layers
Intrinsic amorphous silicon is deposited onto both sides of the substrate using PECVD from a mixture of silane (SiH4) and hydrogen (H2), forming the heterojunction and passivating the surface. Although intrinsic buffer layers are effectively non-conductive, charge carriers can diffuse through as the thickness is typically less than 10 nm. The buffer layer must be sufficiently thick to provide adequate passivation, however must be thin enough to not significantly impede carrier transport or absorb light. It is advantageous for the passivating layer to have a higher band gap to minimise parasitic absorption of photons, as absorption coefficient is partially dependent on band gap. Despite similarities between the buffer layer structure and Metal–Insulator–Semiconductor (MIS) solar cells, SHJ do not necessarily rely on quantum tunnelling for carrier transport through the low-conductivity buffer layer; carrier diffusion is also an important transport mechanism.[11][70]
Window Layers
The selective contacts (also referred to as the "window layers") are then similarly formed by deposition of the p- and n-type highly doped amorphous silicon layers.[71][72] Examples of dopant gases include phosphine (PH3) for n-type and trimethylborane (B(CH3)3) or diborane (B2H6) for p-type.[73] Due to its defective nature, doped amorphous silicon (as opposed to intrinsic) cannot provide passivation to crystalline silicon; similarly epitaxial growth of any such a-Si layer causes severe detriment to passivation quality and cell efficiency and must be prevented during deposition.[74]
Nanocrystalline window layer
Recent developments in SHJ efficiency have been made by deposition of n-type nanocrystalline silicon oxide (nc-SiOx:H) films instead of n-type amorphous silicon for the electron contact. The material commonly referred to as "nanocrystalline silicon oxide" is actually a two-phase material composed of nanoscale silicon crystals embedded in an amorphous silicon oxide matrix. The silicon oxide has a higher band gap and is more optically transparent than amorphous silicon, whereas the columnar nanocrystals enhance vertical carrier transport and increase conductivity, thus leading to increased short circuit current density [math]\displaystyle{ J_{SC} }[/math][66] and decreased contact resistance.[25] The material band gap can be tuned with varying levels of carbon dioxide during PECVD.[75] The replacement of amorphous silicon with nanocrystalline silicon/silicon oxide has already been integrated by some manufacturers on n-type, with p-type (hole contact) to follow in the near future.[27] An optimised nanocrystalline hole contact was instrumental in producing the Lin, et al.. (2023) 26.81% power conversion efficiency world record.[25]
Antireflection coating and conductive oxide
The dual purpose antireflection coating (ARC) and carrier transport layer, usually composed of Indium tin oxide (ITO), is sputtered onto both sides over the selective contacts. Indium tin oxide is a transparent conducting oxide (TCO) which enhances lateral conductivity of the contact surfaces without significantly impeding light transmission. This is necessary because the amorphous layers have a relatively high resistance despite their high doping levels, and so the TCO allows carriers to be transported from the selective contact to the metal electrodes.
For destructive interference antireflection properties, the TCO is deposited to the thickness required for optimum light capture at the peak of the solar spectrum (around 550 nm ). The optimum thickness for a single-layer ARC is given by;
[math]\displaystyle{ d=\frac{\lambda}{4\eta} }[/math]
where [math]\displaystyle{ d }[/math] is the layer thickness, [math]\displaystyle{ \lambda }[/math] is the desired wavelength of minimum reflection and [math]\displaystyle{ \eta }[/math] is the material's refractive index.
Depending on the refractive index of the ITO (typically ~0.9),[76] the optimum layer thickness is usually 70–80 nm. Due to thin-film interference, the ITO (a dull grey-black ceramic material) appears a vibrant blue colour at this thickness.
Alternative materials
Due to the scarcity of indium, alternative TCOs such as aluminium-doped zinc oxide (AZO) are being researched for use in SHJ cells.[77] AZO has a much higher chemical sensitivity than ITO, which presents challenges for certain metallisation methods that require etching, such as nickel seed layer etch-backs[61] and typically has a poorer interface contact to both p- and n-type amorphous layers.[78] AZO may have long-term stability issues when cells are used in modules, which may require capping layers such as SiOx.[79][80]
Undoped tin oxide (SnOx) has also been used successfully to produce indium-free TCOs on SHJ cells with an efficiency of 24.91%.[81]
Enhancement of the optical and electronic properties of indium oxide based TCOs has been achieved through co-doping with cerium and hydrogen, which results in high electron mobility. Such films can be grown at temperatures sufficiently low to be compatible with the heat-sensitive SHJ production process.[82][80] Indium oxide doped with cerium oxide, tantalum oxide and titanium oxide have also resulted in favourable electronic properties. The process is tunable through introduction of water vapour into the sputtering chamber[75] in which hydroxyl radicals in the plasma are believed to terminate oxygen vacancies in the TCO film, leading to enhanced electron mobility and lower sheet resistance, however stability and contact resistance must be considered when using this method in SHJ cells.[83]
Double-antireflection coating
Through evaporation, a double-antireflection coating of magnesium fluoride (MgF2)[84] or aluminium oxide (Al2O3)[76] may be used to further reduce surface reflections, however this step is not currently employed in industrial production. AZO capping layers such as SiOx can also act as a double AR coating.[79] Such techniques were used to produce SHJ cells with world record power conversion efficiencies.[25]
Role of work function
The TCO layer for SHJ cells should ideally have a high work function[85] (ie. the energy difference between the Fermi level and the Vacuum level) to prevent formation of a parasitic Schottky barrier at the interface between the TCO and the p-type amorphous layer.[86][87][88] This can be partially alleviated by increasing the doping of the p-type layer, which decreases the barrier width and improves open-circuit voltage ([math]\displaystyle{ V_{OC} }[/math]) and fill factor ([math]\displaystyle{ FF }[/math]). However increased doping increases junction recombination, diminishing [math]\displaystyle{ V_{OC} }[/math] gains. Depositing a higher work function TCO such as tungsten oxide (WOx) or tuning the deposition parameters of ITO can also reduce the barrier height; typically the latter is used due to the preferable optical properties of ITO.[78]
Metallisation
Metal electrodes are required to contact the solar cell so that electricity can be extracted from it. The TCO alone is not conductive enough to serve this purpose. The electrodes on a bifacial solar cell are composed of a grid pattern on the front side and the rear side, whereas non-bifacial cells can have the entire rear side coated in metal. Interdigitated back contact cells have metal only on the rear. In the case of front grids, the grid geometry is optimised such to provide a low resistance contact to all areas of the solar cell surface without excessively shading it from sunlight.
Printed paste
Heterojunction solar cells are typically metallised (ie. fabrication of the metal contacts) in two distinct methods. Screen-printing of silver paste is common in industry as is with traditional solar cells, with a market share of over 98%.[3] However low-temperature silver paste is required for SHJ cells. These pastes consist of silver particles combined with a polymer which crosslinks at a curing temperature of about 200 °C.[63] These suffer major drawbacks including low grid conductivity and high silver consumption,[62][89] volatile production costs[22] or poor adhesion to the front surface.[14][62] Despite their significantly higher cost,[61] the resistivity of low-temperature silver pastes has been estimated to be 4–6 times higher than standard silver paste.[11] To compensate for lowered conductivity, low-temperature silver pastes also consume more silver than conventional silver pastes,[62] however silver consumption is trending downward as the development of screen-printing technology reduces finger linewidths.[90] Improvements in the composition of low-temperature pastes are expected to further reduce silver consumption, such as through screen-printable silver-coated copper paste. Such pastes perform comparably to conventional low-temperature pastes, with up to 30% reduction in silver consumption.[91] Silver-coated copper pastes are becoming an increasingly dominant metallisation technology amongst Chinese SHJ manufacturers into 2030, with 50% market share expected from 2024 to 2025.[34]
A non-contact method of paste printing, Laser Pattern Transfer Printing, can be used to fabricate narrow fingers with a 1:1 aspect ratio. Paste is pressed into a grating, and an infrared laser is used to heat the paste from behind. The vaporising solvent expels the paste from the mold and onto the solar cell substrate.[92] As contact screen printing exerts high forces on the cell, this alternative technique can reduce cell breakage, in particular for very thin wafers.[93]
Printed ink
Silver nanoparticle ink can be deposited onto a SHJ solar cell using inkjet printing, or through contact deposition with a hollow glass capillary. Inkjet deposition has been reported to reduce silver consumption from 200 mg per cell to less than 10 mg per cell compared with traditional silver paste screen printing. Further reductions are possible with capillary deposition (known as "FlexTrail" as the capillary is flexible and trails across the wafer surface) leading to as little as 3 mg of silver deposited.[91] Such a large reduction in silver has implications for the grid design to compensate for lower conduction, namely using a busbar-less design.
Electroplated

A potentially silver-free[44] alternative to printed electrodes uses electroplated copper. The conductivity of electroplated copper is similar to that of bulk copper.[94] This has potential to increase the SHJ cell current density through decreasing grid resistance. Improved feature geometry can also be achieved. However industrial production is challenging as electroplating requires selective patterning using a sacrificial inkjet-printed or photolithographically-derived mask.[89][95] As a result, electroplated SHJ cells are not currently manufactured commercially. Copper plated directly to the ITO also suffers from adhesion issues. Therefore, it is usually necessary to first deposit a thin (~1μm) seed layer of nickel through sputtering or electrodeposition.[61][62][96][97] Alternatively, an indium seed layer can be developed in-situ through selective cathodic reduction of the doped indium oxide.[98] Nickel and ITO layers also act as a diffusion barrier against copper into the cell, which is a deep-level impurity that causes severe degradation.[64] A capping layer of silver or tin is generally also required to prevent corrosion of the copper fingers, especially in EVA-encapsulated modules.[99]
Like all conventional solar cells, heterojunction solar cells are a diode and conduct current in only one direction. Therefore, for metallisation of the n-type side, the solar cell must generate its own plating current through illumination, rather than using an external power supply. This process is known as Light-induced Plating (LIP); as opposed to field-induced plating (FIP) for the p-type side. Alternatively, an electroless process may be used, which does not require electrical contact to the solar cell that complicates manufacturing. However, electroless plating is much slower than electroplating and may take hours rather than minutes to reach a suitable thickness.[100]
Interconnection
SHJ temperature sensitivity has further implications for cell interconnection when manufacturing SHJ-based solar panels. High temperatures involved in soldering must be carefully controlled to avoid degradation of the cell passivation. Low temperature pastes have also suffered from weak adhesion to interconnecting wires or ribbons, which have consequences for module durability. Optimisation of these pastes and infrared soldering parameters, as well as careful selection of solder alloys, has led to increased success of interconnection processes on standard industrial equipment.[101]
Multi-junction
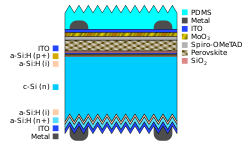
Heterojunction–Perovskite tandem structures have been fabricated, with some research groups reporting a power conversion efficiency exceeding the 29.43% Shockley–Queisser limit for crystalline silicon. This feat has been achieved in both monolithic and 4-terminal cell configurations.[103][29] In such devices, to reduce thermalisation losses, the wide bandgap Perovskite top cell absorbs high energy photons whilst the SHJ bottom cell absorbs lower energy photons. In a bifacial configuration, the bottom cell can also accept light from the rear surface.
In 2017, tandem solar cells using a SHJ bottom cell and Group III–V semiconductor top cells were fabricated with power conversion efficiencies of 32.8% and 35.9% for 2- and 3-junction non-monolithic stacks respectively.[104]
In November 2023, the efficiency record for SHJ tandems was set at 33.9% by LONGi using a Perovskite top cell in a monolithic configuration.[29] This is the highest efficiency recorded for a non-concentrated Two-junction solar cell.
Alternative heterojunction materials
Aside from the typical c-Si/a-Si:H structure, various groups have successfully produced passivated contact silicon heterojunction solar cells using novel semiconducting materials, such as between c-Si/SiOx,[73] c-Si/MoOx[105][106] and c-Si/poly-Si or c-Si/SiOx/poly-Si (POLO; polycrystalline silicon on oxide).[107][108] Hybrid inorganic–organic heterojunction solar cells have been produced using n-type silicon coated with polyaniline emeraldine base.[109] Heterojunction solar cells have also been produced on multicrystalline silicon absorber substrates.[110]
Material | Band gap energy; Eg (eV) | Notes | Reference |
---|---|---|---|
c-Si | ~1.12 | Typical figure measured at 298 K | [1] |
a-Si:H | ~1.7 | Compared to c-Si, the wider band gap is attributable mostly to the high (~10% in SHJ solar cells) hydrogen content of amorphous silicon.[111] The band gap energy is affected by the crystalline fraction and hydrogen content of the amorphous network, and is dependent on the method in which the thin film is prepared. A higher ratio of H2:SiH4 during deposition increases the band gap energy.[112] | [113] |
SiOx:H | ~1.4–3.3 | Band gap increases as oxygen content [math]\displaystyle{ x }[/math] increases where [math]\displaystyle{ 0 \lt x\lt 2 }[/math]. A higher ratio of CO2:SiH4 during deposition increases the band gap energy.[114] | [115] |
MoOx | ~3 | [106] | |
CH3NH3PbX3 | ~1.55–2.3 | Methylammonium Lead Trihalide perovskite. The bandgap is tunable depending on the ratio of different halides, especially between bromine and iodine. Although the optimum band gap for single junction solar cells is 1.1–1.4 eV (based on the Shockley–Queisser limit), perovskites with a higher band gap may be suitable as the top cell in a tandem heterojunction solar cell. | [116] |
H2NCHNH2PbX3 | ~1.48–2.23 | Formamidinium Lead Trihalide perovskite. The bandgap is tunable depending on the ratio of different halides. | [116] |
Interdigitated Back Contact
Heterojunction solar cells are compatible with IBC technology, ie. the cell metallisation is entirely on the back surface. A Heterojunction IBC cell is often abbreviated to HBC. A HBC structure has several advantages over conventional SHJ cells; the major advantage is the elimination of shading from the front grid, which improves light capture and hence short circuit current density [math]\displaystyle{ J_{SC} }[/math]. Compared to PERC, conventional SHJ cells often suffer from poor [math]\displaystyle{ J_{SC} }[/math] with values rarely exceeding 40 mA/cm2, as some light is parasitically absorbed in the front amorphous silicon layers due to its high absorption coefficient.[66] By removing the need for the front metal contact, as well as the front amorphous silicon contact, [math]\displaystyle{ J_{SC} }[/math] can be recovered. As such, HBC cells have potential for high efficiencies; notably a long-standing world record heterojunction cell employed a HBC structure, at 26.7% efficiency fabricated by Kaneka with a [math]\displaystyle{ J_{SC} }[/math] of 42.65 mA/cm2.[24][117] Despite HBC's high efficiency, double-sided cells are mainstream in industrial production due to their relatively simple manufacturing process.[75] However, HBC cells may find specialised applications such as in vehicle-integrated PV systems where there are significant area constraints.[118]
HBC cells are fabricated by localised doping of the rear side, in an alternating pattern of p- and n-type areas in an interdigitated pattern. The front side does not require a specific doping profile.[119]
Loss mechanisms
A well-designed silicon heterojunction module has an expected nominal lifespan of more than 30 years,[12] with an expected average performance ratio of 75%.[44] Failure, power losses and degradation of SHJ cells and modules can be categorised by the affected parameter (eg. open-circuit voltage, short-circuit current and fill factor). [math]\displaystyle{ V_{OC} }[/math] losses are generally attributed to reduction in passivation quality or through introduction of defects, causing increased recombination. [math]\displaystyle{ J_{SC} }[/math] losses are generally attributed to optical losses, in which less light is captured by the absorber (such as through shading or damage to module structures). [math]\displaystyle{ FF }[/math] losses are generally attributed to passivation loss, and increases in series resistance or decreases in shunt resistance.[12]
VOC losses
Defects are sites at which charge carriers can inadvertently become "trapped", making them more likely to recombine through the Shockley-Read-Hall method (SRH Recombination). They are most likely to exist at interfaces (surface recombination), at crystal grain boundaries and dislocations, or at impurities. To prevent losses in efficiency, defects must be passivated (ie. become chemically and electrically neutral). Generally this occurs through bonding of the defect interface with interstitial hydrogen. In SHJ cells, hydrogenated intrinsic amorphous silicon is very effective at passivating defects existing at the absorber surface.
Understanding the behaviour of defects, and how they interact with hydrogen over time and in manufacturing processes, is crucial for maintaining the stability and performance of SHJ solar cells.
Light-induced Degradation
The behaviour of light-sensitive defect passivation in amorphous silicon networks has been a topic of study since the discovery of the Staebler–Wronski effect in 1977.[120] Staebler and Wronski found a gradual decrease in photoconductivity and dark conductivity of amorphous silicon thin films upon exposure to light for several hours. This effect is reversible upon dark annealing at temperatures above 150 °C and is a common example of reversible Light-induced Degradation (LID) in hydrogenated amorphous silicon devices. The introduction of new band gap states, causing a decrease in the carrier lifetime, was proposed to be the mechanism behind the degradation. Subsequent studies have explored the role of hydrogen migration and metastable hydrogen-trapping defects in the Staebler–Wronski effect.[121]
Amongst many variables, the kinetics and extent of the Staebler–Wronski effect is dependent on crystallite grain size in the thin film[122] and the light soaking illuminance.[123]
Some amorphous silicon devices can also observe the opposite effect through LID, such as the increase in [math]\displaystyle{ V_{OC} }[/math] observed in amorphous silicon solar cells[124][125] and notably SHJ solar cells[126] upon light soaking. Kobayashi, et al. (2016) proposes that this is due to the shifting of the Fermi level of the intrinsic buffer layer closer to the band edges when in contact with the doped amorphous silicon selective contacts,[126] noting that a similar reversal of the Staebler–Wronski effect was observed by Scuto et al. (2015) when hydrogenated a-Si photovoltaic devices were light-soaked under reverse bias.[127]
Deliberate annealing of heterojunction cells in an industrial post-processing step can improve lifetimes and decrease surface recombination velocity. It has been suggested that thermal annealing causes interstitial hydrogen to diffuse closer to the heterointerface, allowing greater saturation of dangling bond defects.[128] Such a process may be enhanced using illumination during annealing, however this can cause degradation before the improvement in carrier lifetimes is achieved, and thus requires careful optimisation in a commercial setting.[129] Illuminated annealing at high temperatures is instrumental in the Advanced Hydrogenation Process (AHP), an inline technique for defect mitigation developed by UNSW.
The Boron–Oxygen complex LID defect is a pervasive problem with the efficiency and stability of cheap p-type wafers and a major reason that n-type is preferred for SHJ substrates. Stabilising wafers against B–O LID using the Advanced Hydrogenation Process has had variable success and reliability issues.[55] Therefore gallium has been proposed as an economically feasible alternative p-type dopant for use in SHJ absorbers.[130][54] Gallium doped cells have potential for higher stability and lower defect density than boron, with research groups achieving [math]\displaystyle{ V_{OC} }[/math] exceeding 730 mV on gallium-doped p-type SHJ.[55] However, gallium has a lower segregation efficiency than boron in Cz-grown silicon ingots, therefore having a similar problem to n-type in that less ingot length is used.[56]
FF losses
Fill factor refers to how well the solar cell performs at its maximum power point compared to open- or short-circuit conditions.
Fill factor in high-efficiency solar cells is affected by several key factors: series resistance; bulk carrier lifetimes; the saturation current density; wafer resistivity and wafer thickness. These factors in turn affect the [math]\displaystyle{ V_{OC} }[/math] and the diode ideality factor. To achieve a fill factor over 86%, a high efficiency heterojunction cell must have a very high shunt resistance, a negligible series resistance, high quality bulk silicon with very long minority carrier lifetime (~15 ms), excellent passivation (saturation current density below 0.8 fA/cm2).
The diode ideality factor will approach 2/3 when the bulk wafer lifetimes increase, implying that Auger recombination becomes the dominant mechanism when bulk defect density is very low. An ideality factor of less than 1 will enable fill factors greater than 86%, as long as bulk lifetimes are high. Very high lifetimes are easier to achieve when the wafer thickness is reduced. At sufficiently high lifetimes, it is also advantageous to decrease the bulk doping concentration (increase the wafer resistivity [math]\displaystyle{ \rho }[/math] > 0.3 Ω·cm) such that the wafer is under high injection conditions (the number of generated carriers is high compared to the dopant concentration).[35]
Module degradation
Solar modules are exposed to various stressors when deployed in outdoor installations, including moisture, thermal cycling and ultraviolet light. Solar modules may be expected to be in service for decades, and these factors can reduce module lifespan if unaccounted for. The mechanisms of degradation include efficiency loss in the cell itself from cracking, gradual corrosion or defect activation; delamination of the module layers; UV degradation of the cell or lamination; encapsulant embrittlement or discolouration; and failure of the metal conductors (fingers, busbars and tabbing).[131] Some significant design considerations for module longevity are in encapsulant choice, with significant reductions in the module's levelised cost of electricity (LCOE) for encapsulants with fewer adverse effects on module efficiency.[132]
Potential-induced Degradation
Potential-induced degradation (PID) refers to degradation caused by high voltage stress in solar modules. It is one of the primary mechanisms of solar module degradation.[133] Strings of modules in series can accumulate up to 1000 V in a photovoltaic system, and such a potential difference can be present over a small distance between the solar cells and a grounded module frame, causing leakage currents. PID is primarily an electrochemical process causing corrosion[134] and ion migration[135] in a solar module and cells, facilitated by moisture ingress and surface contamination.[136][137] Sodium ions, which are suspected to leach from soda-lime glass, are particularly problematic and can cause degradation in the presence of moisture (even without high electric potential).[138] This leads to reduction in the efficiency and lifespan of a PV system.
PID has been observed in all types of crystalline silicon solar cells, as well as thin-film solar cells, CIGS cells and CdTe cells. In research, PID can be replicated in accelerated aging tests by applying high bias voltages to a sample module, especially in an environmental chamber. In SHJ cells, PID is mostly characterised by the reduction in [math]\displaystyle{ J_{SC} }[/math] caused by optical losses, and unlike the PID observed in other module technologies, the PID is mostly irreversible in SHJ modules with only a small recovery from applying the opposite bias. This indicates that some component of the PID occurs through a different mechanism in SHJ modules. It has been suggested that optical losses are caused by indium metal precipitating in the TCO. Degraded modules have also measured high concentrations of sodium ions deeper in the cell, which is consistent with PID caused from negative bias.[133]
Encapsulant hydrolysis
Encapsulants are thermoplastic materials used to encase solar cells in modules for stability. In the lamination process, the cells are sandwiched between the encapsulant film and it is melted. Traditionally, the cheap copolymer Ethylene-vinyl acetate (EVA) has been used in crystalline silicon modules as encapsulant.[139] After long duration exposure to moisture, EVA can hydrolyse and leach acetic acid[140] with the potential to corrode the metal terminals[141] or surface[142] of a solar cell.
Non-bifacial modules are composed of a textured glass front and UV-stabilised polymer (commonly polyvinyl fluoride) backsheet, whereas bifacial modules are more likely to be glass–glass.[12] The polymer backsheet, despite being more permeable to moisture ingress than glass–glass modules (which facilitates hydrolysis of EVA), is allegedly "breathable" to acetic acid and does not allow it to build up. As SHJ-based modules are more likely to be bifacial glass–glass, the risk of acetic acid buildup is claimed to be greater;[139] however manufacturers have found the impermeability of glass–glass modules is generally sufficient to prevent EVA degradation, allowing modules to pass accelerated aging tests. Some studies have also found that glass–glass construction reduces the extent of degradation in EVA-encapsulated modules against glass–backsheet.[143]
Additionally, ITO used in SHJ cells may be susceptible to acetic acid etching, causing [math]\displaystyle{ V_{OC} }[/math] loss.[142][144] Despite the higher cost, acetate-free and low water vapour permeability encapsulants such as polyolefin elastomers (POE) or thermoplastic olefins (TPO) show reduced degradation after damp-heat testing in comparison to EVA.[139][143] It has been estimated that using POE or TPO over EVA can reduce the LCOE by nearly 3% as a result of improved module longevity.[132]
Encapsulant-free module designs have also been developed with potential for reduced long term degradation and CO2 footprint. However reflection losses may arise from the lack of optical coupling between the front glass and the cell that encapsulant provides.[145]
Encapsulant delamination
POE has higher resistance to water ingress compared to EVA, and hence prevents PID and other moisture-related issues. However, the lamination time is longer, and the adhesion between POE and the cell or glass is inferior to EVA. Delamination of encapsulant from poor adhesion can cause failure of the module. Therefore, POE is increasingly used as the centre layer in a three-layer coextruded polymer encapsulant with EVA, known as EPE (EVA–POE–EVA) which entails the benefits of both polymers.[146][147]
UV stability
UV light can cause degradation of module encapsulants and backsheets, causing discolouration, embrittlement and delamination that reduces module lifespan and performance. Hot carriers generated by UV absorption can also cause oxidation of such materials. Furthermore, in high efficiency solar cells including heterojunction, UV causes changes in passivation that may decrease module performance. Studies involving extended UV light soaking of heterojunction modules indicate they are more susceptible to UV damage than PERC or PERT modules, where significant losses in fill factor and open-circuit voltages were observed. The proposed mechanism is the redistribution of hydrogen away from the passivated surface interfaces and into the amorphous layers.[148]
UV cut-off encapsulant films have been used to protect SHJ cells from UV degradation, however the UV energy from such materials is not used by the solar cells. In 2023, encapsulant films containing UV down-converting phosphors such as Europium/Dysprosium-doped Strontium magnesium silicate (Sr2-xMgSi2O7-x: Eu2+, Dy3+) were introduced for heterojunction solar cell applications, such as in EPE encapsulants. Such materials not only protect from UV degradation but also deliver optical gains from generated visible photons.[149] Such films are being investigated for commercial use by Chinese heterojunction encapsulant manufacturers where tests of 60-cell modules saw power increases of 5 watts (approximately 1.5%) using the UV-converting film.[150][151]
Glossary
The following is a glossary of terms associated with heterojunction solar cells.
- heterojunction
- A junction between any two materials formed by their dissimilar band gap energies
- selective contact
- A layer of the solar cell (eg. doped amorphous silicon) that discriminates electrons from holes, allowing them to be separated. There are always two such contacts, doped in opposite polarities on the front and rear of the cell (relative to each other) depending on whether holes or electrons are to be collected.
- passivation
- Any phenomenon that reduces the likelihood of charge carriers recombining. Passivation of parasitic defects (eg. contaminant atoms, dangling bonds, crystal grain boundaries) refers to them being electrically neutralised such that they do not "trap" charge carriers, thereby preventing SRH Recombination. It is generally desirable for a solar cell to be well passivated, particularly at the interface between the metallic contact and the semiconductor. Passivation can also be achieved using an electric field, such as in Al-BSF solar cells (field effect passivation).
- hydrogenation
- A technique in which interstitial hydrogen is used to passivate regions of the solar cell
- buffer layer
- A very thin electrically inactive layer, typically intrinsic amorphous silicon, that provides surface passivation for the SHJ substrate
- window layer
- The (ideally) transparent selective contacts of a SHJ cell, typically made from amorphous or nanocrystalline silicon in a very thin layer
- pyramids
- The microscale pyramidal texture on the surface of a solar cell after alkaline isotropic etching procedure. Pyramids decrease solar cell surface reflectivity, allowing more light to be captured.
- metallisation
- The process by which the metal electrodes are formed on the positive and negative regions of the solar cell. These contact the solar cell to extract electricity from it.
References
- ↑ 1.0 1.1 Dupré, Olivier; Vaillon, Rodolphe; Green, Martin A. (2017) (in en). Thermal Behavior of Photovoltaic Devices. Cham: Springer International Publishing. doi:10.1007/978-3-319-49457-9. ISBN 978-3-319-49456-2. http://link.springer.com/10.1007/978-3-319-49457-9.
- ↑ 2.0 2.1 2.2 Bellini, Emiliano (21 November 2022). "Longi claims world's highest efficiency for silicon solar cells". https://pv-magazine-usa.com/2022/11/21/longi-claims-worlds-highest-efficiency-for-silicon-solar-cells/.
- ↑ 3.0 3.1 3.2 3.3 Fischer, Markus; Woodhouse, Michael; Herritsch, Susanne; Trube, Jutta (2022). "International Technology Roadmap for Photovoltaic (ITRPV)" (in en) (13 ed.). Frankfurt, Germany: VDMA e. V. https://itrpv.vdma.org/en/ueber-uns.
- ↑ Lipovšek, Benjamin; Smole, Franc; Topič, Marko; Humar, Iztok; Sinigoj, Anton Rafael (2019-05-01). "Driving forces and charge-carrier separation in p-n junction solar cells" (in en). AIP Advances 9 (5). doi:10.1063/1.5092948. ISSN 2158-3226. https://pubs.aip.org/adv/article/9/5/055026/1070438/Driving-forces-and-charge-carrier-separation-in-p.
- ↑ Green, Martin A. (1982). Solar Cells: Operating Principles, Technology and System Applications. ISBN 9780858235809.
- ↑ Wang, Guangyi; Zhang, Chenxu; Sun, Heng; Huang, Zengguang; Zhong, Sihua (2021-11-01). "Understanding and design of efficient carrier-selective contacts for solar cells" (in en). AIP Advances 11 (11). doi:10.1063/5.0063915. ISSN 2158-3226. https://pubs.aip.org/adv/article/11/11/115026/990219/Understanding-and-design-of-efficient-carrier.
- ↑ Descoeudres, A.; Barraud, L.; De Wolf, Stefaan; Strahm, B.; Lachenal, D.; Guérin, C.; Holman, Z. C.; Zicarelli, F. et al. (2011). "Improved amorphous/crystalline silicon interface passivation by hydrogen plasma treatment" (in en). Applied Physics Letters 99 (12): 123506. doi:10.1063/1.3641899. Bibcode: 2011ApPhL..99l3506D. https://infoscience.epfl.ch/record/169710/files/paper_614.pdf.
- ↑ Olibet, Sara; Vallat-Sauvain, Evelyne; Ballif, Christophe (July 2007). "Model for a-Si:H/c-Si interface recombination based on the amphoteric nature of silicon dangling bonds" (in en). Physical Review B 76 (3): 035326. doi:10.1103/PhysRevB.76.035326. Bibcode: 2007PhRvB..76c5326O. http://infoscience.epfl.ch/record/133997.
- ↑ 9.0 9.1 Taguchi, Mikio; Terakawa, Akira; Maruyama, Eiji; Tanaka, Makoto (2005). "Obtaining a higher VOC in HIT cells" (in en). Progress in Photovoltaics: Research and Applications 13 (6): 481–488. doi:10.1002/pip.646.
- ↑ Zhang, D.; Tavakoliyaraki, A.; Wu, Y.; van Swaaij, R.A.C.M.M.; Zeman, M. (2011). "Influence of ITO deposition and post annealing on HIT solar cell structures" (in en). Energy Procedia 8: 207–213. doi:10.1016/j.egypro.2011.06.125. ISSN 1876-6102.
- ↑ 11.0 11.1 11.2 11.3 11.4 De Wolf, Stefaan; Descoeudres, A.; Holman, Z.C.; Ballif, C. (2012). "High-efficiency Silicon Heterojunction Solar Cells: A Review" (in en). Green 2 (1): 7–24. doi:10.1515/green-2011-0018. ISSN 1869-8778. https://infoscience.epfl.ch/record/269423/files/green-2011-0018.pdf.
- ↑ 12.0 12.1 12.2 12.3 12.4 12.5 Arriaga Arruti, Olatz; Virtuani, Alessandro; Ballif, Christophe (2023-03-02). "Long‐term performance and reliability of silicon heterojunction solar modules" (in en). Progress in Photovoltaics: Research and Applications 31 (7): 664–677. doi:10.1002/pip.3688. ISSN 1062-7995. https://onlinelibrary.wiley.com/doi/10.1002/pip.3688.
- ↑ De Wolf, Stefaan; Kondo, Michio (2009). "Nature of doped a-Si:H/c-Si interface recombination" (in en). Journal of Applied Physics 105 (10): 103707–103707–6. doi:10.1063/1.3129578. Bibcode: 2009JAP...105j3707D. http://infoscience.epfl.ch/record/143812.
- ↑ 14.0 14.1 Descoeudres, A.; Allebé, C. (2018). "Low-temperature processes for passivation and metallization of high-efficiency crystalline silicon solar cells" (in en). Solar Energy 175: 54–59. doi:10.1016/j.solener.2018.01.074. ISSN 0038-092X. Bibcode: 2018SoEn..175...54D.
- ↑ 15.0 15.1 Wright, Matthew; Kim, Moonyong; Dexiang, Peng; Xin, Xu; Wenbin, Zhang; Wright, Brendan; Hallam, Brett (2019). "Multifunctional process to improve surface passivation and carrier transport in industrial n-type silicon heterojunction solar cells by 0.7% absolute". 15th International Conference on Concentrator Photovoltaic Systems (CPV-15). 2149. Fes, Morocco. pp. 110006. doi:10.1063/1.5123882. http://aip.scitation.org/doi/abs/10.1063/1.5123882.
- ↑ Pankove, J.I.; Tarng, M.L. (1979). "Amorphous silicon as a passivant for crystalline silicon" (in en). Applied Physics Letters 34 (2): 156–157. doi:10.1063/1.90711. Bibcode: 1979ApPhL..34..156P.
- ↑ Fuhs, W.; Niemann, K.; Stuke, J. (1974). "Heterojunctions of Amorphous Silicon and Silicon Single Crystals" (in en). AIP Conference Proceedings 20 (1): 345–350. doi:10.1063/1.2945985. Bibcode: 1974AIPC...20..345F.
- ↑ Okuda, Koji; Okamoto, Hiroaki; Hamakawa, Yoshihiro (September 1983). "Amorphous Si/Polycrystalline Si Stacked Solar Cell Having More Than 12% Conversion Efficiency" (in en). Japan Society of Applied Physics 22 (Part 2, No. 9): L605–L607. doi:10.1143/JJAP.22.L605. Bibcode: 1983JaJAP..22L.605O.
- ↑ ; Minami, Kouji & Yamaoki, Toshihiko"Photovoltaic device" US patent expired 5066340, issued 19 November 1991, assigned to Sanyo Electric Co Ltd
- ↑ ; Iwata, Hiroshi & Sano, Keiichi"Photovoltaic device" US patent expired 5213628, issued 25 May 1993, assigned to Sanyo Electric Co Ltd
- ↑ Wang, T.H.; Page, M.R.; Iwaniczko, E. (11 August 2004). "Toward better understanding and improved performance of silicon heterojunction solar cells" (in en). 14th Workshop on Crystalline Silicon Solar Cells and Modules, Winter Park, Colorado, USA, 8–11 August 2004. (National Renewable Energy Lab., Golden, CO (US)). https://www.osti.gov/biblio/15009889. Retrieved 20 August 2020.
- ↑ 22.0 22.1 22.2 22.3 Louwen, Atse; van Sark, Wilfried; Schropp, Ruud; Faaij, André (2016). "A cost roadmap for silicon heterojunction solar cells" (in en). Solar Energy Materials and Solar Cells 147: 295–314. doi:10.1016/j.solmat.2015.12.026. ISSN 0927-0248.
- ↑ Masuko, K.; Shigematsu, M.; Hashiguchi, T.; Fujishima, D. (November 2014). "Achievement of More Than 25% Conversion Efficiency With Crystalline Silicon Heterojunction Solar Cell" (in en). IEEE Journal of Photovoltaics 4 (6): 1433–1435. doi:10.1109/JPHOTOV.2014.2352151.
- ↑ 24.0 24.1 Yamamoto, Kenji; Yoshikawa, Kunta; Uzu, Hisashi; Adachi, Daisuke (July 2018). "High-efficiency heterojunction crystalline Si solar cells" (in en). Japanese Journal of Applied Physics 57 (8S3): 08RB20. doi:10.7567/jjap.57.08rb20. Bibcode: 2018JaJAP..57hRB20Y.
- ↑ 25.0 25.1 25.2 25.3 Lin, Hao; Yang, Miao; Ru, Xiaoning; Wang, Genshun; Yin, Shi; Peng, Fuguo; Hong, Chengjian; Qu, Minghao et al. (2023-05-04). "Silicon heterojunction solar cells with up to 26.81% efficiency achieved by electrically optimized nanocrystalline-silicon hole contact layers" (in en). Nature Energy 8 (8): 789–799. doi:10.1038/s41560-023-01255-2. ISSN 2058-7546. Bibcode: 2023NatEn...8..789L. https://www.nature.com/articles/s41560-023-01255-2.
- ↑ Bhambhani, Anu (20 December 2023). "ISFH Certifies LONGi’s 27.09% Efficiency Claim For HBC Cell". https://taiyangnews.info/longi-new-world-record-efficiency-hbc-solar-cells/.
- ↑ 27.0 27.1 27.2 Chunduri, Shravan (24 March 2023). "Heterojunction Solar Technology 2023 Edition" (in en). TaiyangNews. https://taiyangnews.info/reports/heterojunction-solar-technology-report-2023/.
- ↑ Xiao, Carrie (16 October 2023). "New HJT efficiency and output records in 2023". https://www.pv-tech.org/new-hjt-efficiency-and-output-records-in-2023/.
- ↑ 29.0 29.1 29.2 29.3 29.4 "Best Research-Cell Efficiencies". National Renewable Energy Laboratory. 1 November 2023. https://www.nrel.gov/pv/assets/pdfs/best-research-cell-efficiencies.pdf.
- ↑ Wang, Vera (13 February 2023). "N-type capacity ramps up: Over 900 GW planned by 60 manufacturers". Solarbe Global. https://www.solarbeglobal.com/n-type-capacity-ramps-up-over-900-gw-announced-by-60-manufacturers/.
- ↑ "129GW Inventory of HJT cell expansion projects in 2022: Huasun, Longi, Golden Glass, Risen, Mingyang Smart, Akcome, CR Power; equipment manufacturers usher in huge market opportunities". 19 August 2022. http://www.asiachem.org/en/pv_hjt1.
- ↑ "Huasun high efficiency HJT modules contribute to Bulgarian energy transformation" (in en-US). 2022-04-15. https://www.pv-tech.org/industry-updates/huasun-high-efficiency-hjt-modules-contribute-to-bulgarian-energy-transformation/.
- ↑ Norman, Will (11 April 2023). "Huasun inks 1.5GW HJT module supply deal with Bulgarian EPC firm Inercom" (in en-US). https://www.pv-tech.org/huasun-inks-1-5gw-hjt-module-supply-deal-with-bulgarian-epc-firm-inercom/.
- ↑ 34.0 34.1 34.2 "中国光伏产业发展路线图 (2022–2023)" (in zh). 16 February 2023. http://www.chinapv.org.cn/downf/pdf/file/filea/id/1137.html.
- ↑ 35.0 35.1 Razzaq, Arsalan; Allen, Thomas G.; De Wolf, Stefaan (2023-09-29). "Design Criteria for Silicon Solar Cells with Fill Factors Approaching the Auger Limit" (in en). ACS Energy Letters: 4438–4440. doi:10.1021/acsenergylett.3c01519. ISSN 2380-8195. https://pubs.acs.org/doi/10.1021/acsenergylett.3c01519.
- ↑ Yin, H.P.; Zhou, Y.F.; Sun, S.L.; Tang, W.S.; Shan, W.; Huang, X.M.; Shen, X.D. (March 2021). "Optical enhanced effects on the electrical performance and energy yield of bifacial PV modules" (in en). Solar Energy 217: 245–252. doi:10.1016/j.solener.2021.02.004. Bibcode: 2021SoEn..217..245Y. https://linkinghub.elsevier.com/retrieve/pii/S0038092X21001031.
- ↑ Sinha, Archana; Sulas-Kern, Dana B; Owen-Bellini, Michael; Spinella, Laura; Uličná, Soňa; Ayala Pelaez, Silvana; Johnston, Steve; Schelhas, Laura T (2021-10-14). "Glass/glass photovoltaic module reliability and degradation: a review". Journal of Physics D: Applied Physics 54 (41): 413002. doi:10.1088/1361-6463/ac1462. ISSN 0022-3727. Bibcode: 2021JPhD...54O3002S. https://iopscience.iop.org/article/10.1088/1361-6463/ac1462.
- ↑ Fischer, Markus; Ni, Paul; Metz, Axel; Erfurt, Gunter; Li, Chi-Chun; Woodhouse, Michael; Xing, Guoqiang; Saha, Ivan et al. (2023). "International Technology Roadmap for Photovoltaic (ITRPV)" (in en) (14 ed.). Frankfurt, Germany: VDMA e. V. https://www.vdma.org/international-technology-roadmap-photovoltaic.
- ↑ Bellini, Emiliano (27 September 2022). "Enel unveils 680 W n-type heterojunction solar panel for utility-scale applications" (in en-AU). https://www.pv-magazine-australia.com/2022/09/27/enel-unveils-680-w-n-type-heterojunction-solar-panel-for-utility-scale-applications/.
- ↑ Haschke, Jan; Seif, Johannes P.; Riesen, Yannick; Tomasi, Andrea; Cattin, Jean; Tous, Loïc; Choulat, Patrick; Aleman, Monica et al. (2017). "The impact of silicon solar cell architecture and cell interconnection on energy yield in hot & sunny climates" (in en). Energy & Environmental Science 10 (5): 1196–1206. doi:10.1039/C7EE00286F. ISSN 1754-5692. http://xlink.rsc.org/?DOI=C7EE00286F.
- ↑ Green, M. A.; Blakers, A. W.; Osterwald, C. R. (December 1985). "Characterization of high‐efficiency silicon solar cells" (in en). Journal of Applied Physics 58 (11): 4402–4408. doi:10.1063/1.336286. ISSN 0021-8979. Bibcode: 1985JAP....58.4402G. http://aip.scitation.org/doi/10.1063/1.336286.
- ↑ Green, M.A.; Emery, K.; Blakers, A.W. (1982). "Silicon solar cells with reduced temperature sensitivity" (in en). Electronics Letters 18 (2): 97. doi:10.1049/el:19820066. Bibcode: 1982ElL....18...97G. https://digital-library.theiet.org/content/journals/10.1049/el_19820066.
- ↑ 43.0 43.1 Leu, Sylvère; Sontag, Detlef (2020), Shah, Arvind, ed., "Crystalline Silicon Solar Cells: Heterojunction Cells" (in en), Solar Cells and Modules (Cham: Springer International Publishing) 301: pp. 163–195, doi:10.1007/978-3-030-46487-5_7, ISBN 978-3-030-46485-1, http://link.springer.com/10.1007/978-3-030-46487-5_7, retrieved 2023-04-17
- ↑ 44.0 44.1 44.2 Louwen, A.; van Sark, W.G.J.H.M.; Schropp, R.E.I.; Turkenburg, W.C.; Faaij, A.P.C. (October 2015). "Life-cycle greenhouse gas emissions and energy payback time of current and prospective silicon heterojunction solar cell designs: LCA of current and prospective silicon heterojunction solar cells" (in en). Progress in Photovoltaics: Research and Applications 23 (10): 1406–1428. doi:10.1002/pip.2540. https://onlinelibrary.wiley.com/doi/10.1002/pip.2540.
- ↑ 45.0 45.1 Liu, Zhe; Sofia, Sarah E.; Laine, Hannu S.; Woodhouse, Michael; Wieghold, Sarah; Peters, Ian Marius; Buonassisi, Tonio (2020). "Revisiting thin silicon for photovoltaics: a technoeconomic perspective" (in en). Energy & Environmental Science 13 (1): 12–23. doi:10.1039/C9EE02452B. ISSN 1754-5692. http://xlink.rsc.org/?DOI=C9EE02452B.
- ↑ Berney Needleman, David; Augusto, Andre; Peral, Ana; Bowden, Stuart; del Canizo, Carlos; Buonassisi, Tonio (June 2016). "Thin absorbers for defect-tolerant solar cell design". 2016 IEEE 43rd Photovoltaic Specialists Conference (PVSC). IEEE. pp. 0606–0610. doi:10.1109/PVSC.2016.7749669. ISBN 978-1-5090-2724-8. https://ieeexplore.ieee.org/document/7749669.
- ↑ Terheiden, Barbara; Ballmann, Tabitha; Horbelt, Renate; Schiele, Yvonne; Seren, Sabine; Ebser, Jan; Hahn, G.; Mertens, Verena et al. (January 2015). "Manufacturing 100-µm-thick silicon solar cells with efficiencies greater than 20% in a pilot production line: Manufacturing 100-µm-thick Si solar cells with efficiencies greater than 20%" (in en). Physica Status Solidi A 212 (1): 13–24. doi:10.1002/pssa.201431241. https://onlinelibrary.wiley.com/doi/10.1002/pssa.201431241.
- ↑ Sai, Hitoshi; Oku, Toshiki; Sato, Yoshiki; Tanabe, Mayumi; Matsui, Takuya; Matsubara, Koji (December 2019). "Potential of very thin and high‐efficiency silicon heterojunction solar cells" (in en). Progress in Photovoltaics: Research and Applications 27 (12): 1061–1070. doi:10.1002/pip.3181. ISSN 1062-7995. https://onlinelibrary.wiley.com/doi/10.1002/pip.3181.
- ↑ 49.0 49.1 Augusto, A.; Karas, J.; Balaji, P.; Bowden, S. G.; King, R. R. (2020). "Exploring the practical efficiency limit of silicon solar cells using thin solar-grade substrates" (in en). Journal of Materials Chemistry A 8 (32): 16599–16608. doi:10.1039/D0TA04575F. ISSN 2050-7488. http://xlink.rsc.org/?DOI=D0TA04575F.
- ↑ Sai, Hitoshi; Umishio, Hiroshi; Matsui, Takuya (November 2021). "Very Thin (56 μm) Silicon Heterojunction Solar Cells with an Efficiency of 23.3% and an Open‐Circuit Voltage of 754 mV" (in en). Solar RRL 5 (11): 2100634. doi:10.1002/solr.202100634. ISSN 2367-198X. https://onlinelibrary.wiley.com/doi/10.1002/solr.202100634.
- ↑ Woodhouse, Michael A.; Smith, Brittany; Ramdas, Ashwin; Margolis, Robert M. (2019-02-15) (in en). Crystalline Silicon Photovoltaic Module Manufacturing Costs and Sustainable Pricing: 1H 2018 Benchmark and Cost Reduction Road Map (Report). doi:10.2172/1495719. http://www.osti.gov/servlets/purl/1495719/.
- ↑ 52.0 52.1 52.2 52.3 Razzaq, Arsalan; Allen, Thomas G.; Liu, Wenzhu; Liu, Zhengxin; De Wolf, Stefaan (March 2022). "Silicon heterojunction solar cells: Techno-economic assessment and opportunities" (in en). Joule 6 (3): 514–542. doi:10.1016/j.joule.2022.02.009. https://linkinghub.elsevier.com/retrieve/pii/S2542435122000903.
- ↑ Chunduri, Shravan (7 Feb 2021). "Suppliers Of Core Layer Deposition Tools For HJT". Taiyang News. https://taiyangnews.info/suppliers-of-core-layer-deposition-tools-for-hjt/.
- ↑ 54.0 54.1 54.2 Chang, Nathan L.; Wright, Matthew; Egan, Renate; Hallam, Brett (June 2020). "The Technical and Economic Viability of Replacing n-type with p-type Wafers for Silicon Heterojunction Solar Cells" (in en). Cell Reports Physical Science 1 (6): 100069. doi:10.1016/j.xcrp.2020.100069. Bibcode: 2020CRPS....100069C. https://linkinghub.elsevier.com/retrieve/pii/S2666386420300643.
- ↑ 55.0 55.1 55.2 Vicari Stefani, Bruno; Kim, Moonyong; Wright, Matthew; Soeriyadi, Anastasia; Andronikov, Dmitriy; Nyapshaev, Ilya; Abolmasov, Sergey; Emtsev, Konstantin et al. (September 2021). "Stability Study of Silicon Heterojunction Solar Cells Fabricated with Gallium‐ and Boron‐Doped Silicon Wafers" (in en). Solar RRL 5 (9): 2100406. doi:10.1002/solr.202100406. ISSN 2367-198X. https://onlinelibrary.wiley.com/doi/10.1002/solr.202100406.
- ↑ 56.0 56.1 Yu, Xuegong; Yang, Deren (2019), Yang, Deren, ed., "Growth of Crystalline Silicon for Solar Cells: Czochralski Si" (in en), Handbook of Photovoltaic Silicon (Berlin, Heidelberg: Springer Berlin Heidelberg): pp. 129–174, doi:10.1007/978-3-662-56472-1_12, ISBN 978-3-662-56471-4, http://link.springer.com/10.1007/978-3-662-56472-1_12, retrieved 2023-07-24
- ↑ Chunduri, Shravan (16 December 2020). "Breakdown of Higher Costs n-type Wafers". https://taiyangnews.info/technology/breakdown-of-higher-costs-n-type-wafers/.
- ↑ Zubel, Irena; Kramkowska, Małgorzata (October 2002). "The effect of alcohol additives on etching characteristics in KOH solutions" (in en). Sensors and Actuators A: Physical 101 (3): 255–261. doi:10.1016/S0924-4247(02)00265-0. https://linkinghub.elsevier.com/retrieve/pii/S0924424702002650.
- ↑ 59.0 59.1 Strinitz, Frank; El Jaouhari, Ahmed; Schoerg, Florian; Fuerst, Martina; Plettig, Martin; Kuehnlein, Holger (September 2017). "Advanced alkaline texturing and cleaning for PERC and SHJ solar cells" (in en). Energy Procedia 130: 23–30. doi:10.1016/j.egypro.2017.09.409. https://linkinghub.elsevier.com/retrieve/pii/S1876610217345009.
- ↑ Morales-Vilches, Anna Belen; Wang, Er-Chien; Henschel, Tobias; Kubicki, Matthias; Cruz, Alexandros; Janke, Stefan; Korte, Lars; Schlatmann, Rutger et al. (February 2020). "Improved Surface Passivation by Wet Texturing, Ozone‐Based Cleaning, and Plasma‐Enhanced Chemical Vapor Deposition Processes for High‐Efficiency Silicon Heterojunction Solar Cells" (in en). Physica Status Solidi A 217 (4): 1900518. doi:10.1002/pssa.201900518. ISSN 1862-6300. Bibcode: 2020PSSAR.21700518M. https://onlinelibrary.wiley.com/doi/10.1002/pssa.201900518.
- ↑ 61.0 61.1 61.2 61.3 Lachowicz, A.; Christmann, G.; Descoeudres, A.; Nicolay, S.; Ballif, C. (2020). "Silver- and Indium-Free Silicon Heterojunction Solar Cell" (in en). 37th European Photovoltaic Solar Energy Conference and Exhibition; 490–492: 3 pages, 1940 kb. doi:10.4229/EUPVSEC20202020-2DV.3.8. https://www.eupvsec-proceedings.com/proceedings?paper=49089.
- ↑ 62.0 62.1 62.2 62.3 62.4 Geissbühler, J.; De Wolf, S.; Faes, A.; Badel, N.; Jeangros, Q.; Tomasi, A.; Barraud, L.; Descoeudres, A. et al. (July 2014). "Silicon Heterojunction Solar Cells With Copper-Plated Grid Electrodes: Status and Comparison With Silver Thick-Film Techniques" (in en). IEEE Journal of Photovoltaics 4 (4): 1055–1062. doi:10.1109/JPHOTOV.2014.2321663.
- ↑ 63.0 63.1 Erath, Denis; Pingel, Sebastian; Khotimah, Retno; De Rose, Angela; Eberlein, Dirk; Wenzel, Timo; Roder, Sebastian; Lorenz, Andreas et al. (1 June 2021). "Fast screen printing and curing process for silicon heterojunction solar cells". 9th Workshop on Metallization and Interconnection For Crystalline Silicon Solar Cells. 2367. Genk, Belgium: AIP Conference Proceedings. doi:10.1063/5.0056429. https://pubs.aip.org/aip/acp/article/610406. Retrieved 2023-09-26.
- ↑ 64.0 64.1 Yu, Jian; Li, Junjun; Zhao, Yilin; Lambertz, Andreas; Chen, Tao; Duan, Weiyuan; Liu, Wenzhu; Yang, Xinbo et al. (2021-06-01). "Copper metallization of electrodes for silicon heterojunction solar cells: Process, reliability and challenges" (in en). Solar Energy Materials and Solar Cells 224: 110993. doi:10.1016/j.solmat.2021.110993. ISSN 0927-0248. https://www.sciencedirect.com/science/article/pii/S0927024821000374.
- ↑ Martinez, Sylvia Leyva; Bernard, Annie Rabi; Chopra, Sagar (December 2022). Solar: What to watch for in 2023 – Solar technology and market update (Report). Wood Mackenzie. https://go.woodmac.com/l/131501/2023-01-17/2ymcw2/131501/16740149002Iug6OHB/US_Solar_Tech_2023_Outlook.pdf.
- ↑ 66.0 66.1 66.2 Haschke, Jan; Dupré, Olivier; Boccard, Mathieu; Ballif, Christophe (December 2018). "Silicon heterojunction solar cells: Recent technological development and practical aspects – from lab to industry" (in en). Solar Energy Materials and Solar Cells 187: 140–153. doi:10.1016/j.solmat.2018.07.018. https://linkinghub.elsevier.com/retrieve/pii/S0927024818303805.
- ↑ Bivour, Martin; Schröer, Sebastian; Hermle, Martin; Glunz, Stefan W. (2014-03-01). "Silicon heterojunction rear emitter solar cells: Less restrictions on the optoelectrical properties of front side TCOs" (in en). Solar Energy Materials and Solar Cells 122: 120–129. doi:10.1016/j.solmat.2013.11.029. ISSN 0927-0248. https://www.sciencedirect.com/science/article/pii/S0927024813006132.
- ↑ Khokhar, Muhammad Quddamah; Hussain, Shahzada Qamar; Kim, Sangho; Lee, Sunhwa; Pham, Duy Phong; Kim, Youngkuk; Cho, Eun-Chel; Yi, Junsin (2020-04-01). "Review of Rear Emitter Silicon Heterojunction Solar Cells" (in en). Transactions on Electrical and Electronic Materials 21 (2): 138–143. doi:10.1007/s42341-020-00172-5. ISSN 2092-7592. https://doi.org/10.1007/s42341-020-00172-5.
- ↑ Hallam, Brett; Chen, Daniel; Kim, Moonyong; Stefani, Bruno; Hoex, Bram; Abbott, Malcolm; Wenham, Stuart (July 2017). "The role of hydrogenation and gettering in enhancing the efficiency of next‐generation Si solar cells: An industrial perspective" (in en). Physica Status Solidi A 214 (7): 1700305. doi:10.1002/pssa.201700305. ISSN 1862-6300. Bibcode: 2017PSSAR.21400305H. https://onlinelibrary.wiley.com/doi/10.1002/pssa.201700305.
- ↑ Taguchi, Mikio; Maruyama, Eiji; Tanaka, Makoto (2008-02-15). "Temperature Dependence of Amorphous/Crystalline Silicon Heterojunction Solar Cells" (in en). Japanese Journal of Applied Physics 47 (2): 814–818. doi:10.1143/JJAP.47.814. ISSN 0021-4922. Bibcode: 2008JaJAP..47..814T. https://iopscience.iop.org/article/10.1143/JJAP.47.814.
- ↑ Zhang, Yue; Yu, Cao; Yang, Miao; He, Yongcai; Zhang, Linrui; Zhang, Jinyan; Xu, Xixiang; Zhang, Yongzhe et al. (2017). "Optimization of the window layer in large area silicon heterojunction solar cells" (in en). RSC Advances 7 (15): 9258–9263. doi:10.1039/C6RA26342A. ISSN 2046-2069. Bibcode: 2017RSCAd...7.9258Z. http://xlink.rsc.org/?DOI=C6RA26342A.
- ↑ Balaji, Pradeep; Augusto, André (2017). "Silicon Heterojunction Solar Cells". https://pv-manufacturing.org/silicon-heterojunction-solar-cells/.
- ↑ 73.0 73.1 Ding, Kaining; Aeberhard, Urs; Finger, Friedhelm; Rau, Uwe (May 2012). "Silicon heterojunction solar cell with amorphous silicon oxide buffer and microcrystalline silicon oxide contact layers" (in en). Physica Status Solidi RRL 6 (5): 193–195. doi:10.1002/pssr.201206030. Bibcode: 2012PSSRR...6..193D. https://onlinelibrary.wiley.com/doi/10.1002/pssr.201206030.
- ↑ Kondo, Michio; De Wolf, Stefaan; Fujiwara, Hiroyuki (2008). "Understanding of Passivation Mechanism in Heterojunction c-Si Solar Cells" (in en). MRS Proceedings 1066: 1066–A03–01. doi:10.1557/PROC-1066-A03-01. ISSN 0272-9172. http://link.springer.com/10.1557/PROC-1066-A03-01.
- ↑ 75.0 75.1 75.2 Tang, Tianwei; Yu, Cao; Peng, Chen‐Wei; Dong, Gangqiang; He, Chenran; Ran, Xiaochao; Jiang, Hao; Allen, Vince et al. (2022-10-30). "Achievement of 25.54% power conversion efficiency by optimization of current losses at the front side of silicon heterojunction solar cells" (in en). Progress in Photovoltaics: Research and Applications 31 (5): 449–460. doi:10.1002/pip.3641. ISSN 1062-7995. https://onlinelibrary.wiley.com/doi/10.1002/pip.3641.
- ↑ 76.0 76.1 Zahid, Muhammad Aleem; Khokhar, Muhammad Quddamah; Cui, Ziyang; Park, Hyeonggi; Yi, Junsin (September 2021). "Improved optical and electrical properties for heterojunction solar cell using Al2O3/ITO double-layer anti-reflective coating" (in en). Results in Physics 28: 104640. doi:10.1016/j.rinp.2021.104640. Bibcode: 2021ResPh..2804640Z. https://linkinghub.elsevier.com/retrieve/pii/S2211379721007312.
- ↑ Meza, Daniel; Cruz, Alexandros; Morales-Vilches, Anna Belen; Korte, Lars; Stannowski, Bernd (February 2019). "Aluminum-Doped Zinc Oxide as Front Electrode for Rear Emitter Silicon Heterojunction Solar Cells with High Efficiency" (in en). Applied Sciences 9 (5): 862. doi:10.3390/app9050862.
- ↑ 78.0 78.1 Ritzau, Kurt-Ulrich; Bivour, Martin; Schröer, Sebastian; Steinkemper, Heiko; Reinecke, Patrick; Wagner, Florian; Hermle, Martin (December 2014). "TCO work function related transport losses at the a-Si:H/TCO-contact in SHJ solar cells" (in en). Solar Energy Materials and Solar Cells 131: 9–13. doi:10.1016/j.solmat.2014.06.026. https://linkinghub.elsevier.com/retrieve/pii/S0927024814003353.
- ↑ 79.0 79.1 Morales-Vilches, Anna B.; Cruz, Alexandros; Pingel, Sebastian; Neubert, Sebastian; Mazzarella, Luana; Meza, Daniel; Korte, Lars; Schlatmann, Rutger et al. (January 2019). "ITO-Free Silicon Heterojunction Solar Cells With ZnO:Al/SiO 2 Front Electrodes Reaching a Conversion Efficiency of 23%". IEEE Journal of Photovoltaics 9 (1): 34–39. doi:10.1109/JPHOTOV.2018.2873307. ISSN 2156-3381. https://ieeexplore.ieee.org/document/8493365.
- ↑ 80.0 80.1 Tutsch, Leonard; Sai, Hitoshi; Matsui, Takuya; Bivour, Martin; Hermle, Martin; Koida, Takashi (July 2021). "The sputter deposition of broadband transparent and highly conductive cerium and hydrogen co‐doped indium oxide and its transfer to silicon heterojunction solar cells" (in en). Progress in Photovoltaics: Research and Applications 29 (7): 835–845. doi:10.1002/pip.3388. ISSN 1062-7995. https://onlinelibrary.wiley.com/doi/10.1002/pip.3388.
- ↑ Yu, Cao; Zou, Qiaojiao; Wang, Qi; Zhao, Yu; Ran, Xiaochao; Dong, Gangqiang; Peng, Chen-Wei; Allen, Vince et al. (2023-08-07). "Silicon solar cell with undoped tin oxide transparent electrode" (in en). Nature Energy: 1–7. doi:10.1038/s41560-023-01331-7. ISSN 2058-7546. https://www.nature.com/articles/s41560-023-01331-7.
- ↑ Koida, Takashi; Ueno, Yuko; Shibata, Hajime (April 2018). "In2O3-Based Transparent Conducting Oxide Films with High Electron Mobility Fabricated at Low Process Temperatures" (in en). Physica Status Solidi A 215 (7): 1700506. doi:10.1002/pssa.201700506. Bibcode: 2018PSSAR.21500506K. https://onlinelibrary.wiley.com/doi/10.1002/pssa.201700506.
- ↑ Wang, Jianqiang; Meng, Chuncai; Zhao, Lei; Wang, Wenjing; Xu, Xixiang; Zhang, Yongzhe; Yan, Hui (July 2020). "Effect of residual water vapor on the performance of indium tin oxide film and silicon heterojunction solar cell" (in en). Solar Energy 204: 720–725. doi:10.1016/j.solener.2020.04.086. Bibcode: 2020SoEn..204..720W. https://linkinghub.elsevier.com/retrieve/pii/S0038092X20304709.
- ↑ Duan, Weiyuan; Lambertz, Andreas; Bittkau, Karsten; Qiu, Kaifu; Rau, Uwe; Ding, Kaining (2022). "A route towards high-efficiency silicon heterojunction solar cells" (in en). Progress in Photovoltaics: Research and Applications 30 (4): 384–392. doi:10.1002/pip.3493.
- ↑ Centurioni, E.; Iencinella, D. (March 2003). "Role of front contact work function on amorphous silicon/crystalline silicon heterojunction solar cell performance". IEEE Electron Device Letters 24 (3): 177–179. doi:10.1109/LED.2003.811405. ISSN 0741-3106. Bibcode: 2003IEDL...24..177C. https://ieeexplore.ieee.org/document/1202519.
- ↑ Kim, Sunbo; Jung, Junhee; Lee, Youn-Jung; Ahn, Shihyun; Hussain, Shahzada Qamar; Park, Jinjoo; Song, Bong-Shik; Han, Sangmyeng et al. (October 2014). "Role of double ITO/In2O3 layer for high efficiency amorphous/crystalline silicon heterojunction solar cells" (in en). Materials Research Bulletin 58: 83–87. doi:10.1016/j.materresbull.2014.05.003. https://linkinghub.elsevier.com/retrieve/pii/S0025540814002608.
- ↑ Hussain, Shahzada Qamar; Oh, Woong-Kyo; Ahn, ShiHyun; Le, Anh Huy Tuan; Kim, Sunbo; Lee, Youngseok; Yi, Junsin (March 2014). "RF magnetron sputtered indium tin oxide films with high transmittance and work function for a-Si:H/c-Si heterojunction solar cells" (in en). Vacuum 101: 18–21. doi:10.1016/j.vacuum.2013.07.004. Bibcode: 2014Vacuu.101...18H. https://linkinghub.elsevier.com/retrieve/pii/S0042207X13002212.
- ↑ Rached, D.; Mostefaoui, R. (June 2008). "Influence of the front contact barrier height on the Indium Tin Oxide/hydrogenated p-doped amorphous silicon heterojunction solar cells" (in en). Thin Solid Films 516 (15): 5087–5092. doi:10.1016/j.tsf.2008.02.031. Bibcode: 2008TSF...516.5087R. https://linkinghub.elsevier.com/retrieve/pii/S0040609008002496.
- ↑ 89.0 89.1 Rohit, Rukmangada; Rodofili, Andreas; Cimiotti, Gisela; Bartsch, Jonas; Glatthaar, Markus (April 2017). "Selective plating concept for silicon heterojunction solar cell metallization" (in en). 7th International Conference on Silicon Photovoltaics, SiliconPV 2017. 124. Freiburg, Germany. pp. 901–906. doi:10.1016/j.egypro.2017.09.289.
- ↑ Lorenz, A.; Klawitter, M.; Linse, M.; Ney, L.; Tepner, S.; Pingel, S.; Sabet, M.S.; Reiner, J. et al. (2022). "Rotary Screen Printed Metallization of Heterojunction Solar Cells: Toward High-Throughput Production with Very Low Silver Laydown" (in en). Energy Technology 10 (8): 2200377. doi:10.1002/ente.202200377.
- ↑ 91.0 91.1 Schube, Jörg; Fellmeth, Tobias; Jahn, Mike; Keding, Roman; Glunz, Stefan W. (2019). "Advanced metallization with low silver consumption for silicon heterojunction solar cells". International Symposium on Green and Sustainable Technology (Isgst2019). 2157. Perak, Malaysia. pp. 020007. doi:10.1063/1.5125872. http://aip.scitation.org/doi/abs/10.1063/1.5125872.
- ↑ Adrian, Adrian (18 May 2022). Deposition of Ultrafine Lines using Pattern Transfer Printing for Metallization of Silicon Solar Cells (PhD of Engineering thesis). Karlsruhe Institute of Technology.
- ↑ Chunduri, Shravan (17 May 2023). "Laser Pattern Transfer Printing And Copper Plating Are 2 Promising Approaches For Improving Metallization Of HJT Cells". TaiyangNews. https://taiyangnews.info/technology/advancements-in-hjt-metallization/.
- ↑ Zeng, Yulian; Peng, Chen-Wei; Hong, Wei; Wang, Shan; Yu, Cao; Zou, Shuai; Su, Xiaodong (October 2022). "Review on Metallization Approaches for High-Efficiency Silicon Heterojunction Solar Cells" (in en). Transactions of Tianjin University 28 (5): 358–373. doi:10.1007/s12209-022-00336-9. ISSN 1006-4982. https://link.springer.com/10.1007/s12209-022-00336-9.
- ↑ Li, Zhongtian; Hsiao, Pei-Chieh; Zhang, Wei; Chen, Ran; Yao, Yu; Papet, Pierre; Lennon, Alison (2015). "Patterning for Plated Heterojunction Cells" (in en). Energy Procedia 67: 76–83. doi:10.1016/j.egypro.2015.03.290.
- ↑ Papet, P.; Hermans, J.; Söderström, T.; Cucinelli, M.; Andreetta, L.; Bätzner, D.; Frammelsberger, W.; Lachenal, D. et al. (2013). "Heterojunction Solar Cells with Electroplated Ni/Cu Front Electrode" (in en). 28th European Photovoltaic Solar Energy Conference and Exhibition; 1976–1979: 4 pages, 4135 kb. doi:10.4229/28THEUPVSEC2013-2DV.3.51. http://www.eupvsec-proceedings.com/proceedings?paper=24963.
- ↑ Hernández, J.L.; Adachi, D.; Schroos, D.; Valckx, N.; Menou, N.; Uto, T.; Hino, M.; Kanematsu, M. et al. (2013). "High Efficiency Copper Electroplated Heterojunction Solar Cells and Modules – The Path towards 25% Cell Efficiency" (in en). 28th European Photovoltaic Solar Energy Conference and Exhibition; 741–743: 3 pages, 1488 kb. doi:10.4229/28THEUPVSEC2013-2AO.2.1. http://www.eupvsec-proceedings.com/proceedings?paper=23544.
- ↑ Li, Junjun; Yu, Jian; Chen, Tao; Zhang, Haichuan; Wang, Qiyun; Wang, Pu; Huang, Yuelong (January 2020). "In-situ formation of indium seed layer for copper metallization of silicon heterojunction solar cells" (in en). Solar Energy Materials and Solar Cells 204: 110243. doi:10.1016/j.solmat.2019.110243. https://linkinghub.elsevier.com/retrieve/pii/S0927024819305720.
- ↑ Wang, Xi (2017). Cost-effective and Reliable Copperplated Metallisation for Silicon Solar Cells: A Development Path (Thesis thesis). UNSW Sydney. doi:10.26190/unsworks/19844.
- ↑ Lennon, Alison; Yao, Yu; Wenham, Stuart (November 2013). "Evolution of metal plating for silicon solar cell metallisation: Silicon solar cell metallisation" (in en). Progress in Photovoltaics: Research and Applications 21 (7): 1454–1468. doi:10.1002/pip.2221. https://onlinelibrary.wiley.com/doi/10.1002/pip.2221.
- ↑ De Rose, A.; Geipel, T.; Eberlein, D.; Kraft, A.; Nowottnick, M. (2019). "Interconnection of Silicon Heterojunction Solar Cells by Infrared Soldering – Solder Joint Analysis and Temperature Study" (in en). 36th European Photovoltaic Solar Energy Conference and Exhibition; 229–234: 6 pages, 8761 kb. doi:10.4229/EUPVSEC20192019-2CO.11.4. https://www.eupvsec-proceedings.com/proceedings?paper=48010.
- ↑ Zheng, Jianghui; Duan, Weiyuan; Guo, Yuzhen; Zhao, Zijun C.; Yi, Haimang; Ma, Fa-Jun; Granados Caro, Laura; Yi, Chuqi et al. (2023). "Efficient monolithic perovskite–Si tandem solar cells enabled by an ultra-thin indium tin oxide interlayer" (in en). Energy & Environmental Science 16 (3): 1223–1233. doi:10.1039/D2EE04007G. ISSN 1754-5692. http://xlink.rsc.org/?DOI=D2EE04007G.
- ↑ Kim, Sangho; Trinh, Thanh Thuy; Park, Jinjoo; Pham, Duy Phong; Lee, Sunhwa; Do, Huy Binh; Dang, Nam Nguyen; Dao, Vinh-Ai et al. (30 July 2021). "Over 30% efficiency bifacial 4-terminal perovskite-heterojunction silicon tandem solar cells with spectral albedo" (in en). Scientific Reports 11 (1): 15524. doi:10.1038/s41598-021-94848-4. ISSN 2045-2322. PMID 34330966.
- ↑ Essig, Stephanie; Allebé, Christophe; Remo, Timothy; Geisz, John F.; Steiner, Myles A.; Horowitz, Kelsey; Barraud, Loris; Ward, J. Scott et al. (2017-08-25). "Raising the one-sun conversion efficiency of III–V/Si solar cells to 32.8% for two junctions and 35.9% for three junctions" (in en). Nature Energy 2 (9): 17144. doi:10.1038/nenergy.2017.144. ISSN 2058-7546. Bibcode: 2017NatEn...217144E. https://www.nature.com/articles/nenergy2017144.
- ↑ Battaglia, Corsin; de Nicolás, Silvia Martín; De Wolf, Stefaan; Yin, Xingtian; Zheng, Maxwell; Ballif, Christophe; Javey, Ali (2014-03-17). "Silicon heterojunction solar cell with passivated hole selective MoO x contact" (in en). Applied Physics Letters 104 (11): 113902. doi:10.1063/1.4868880. ISSN 0003-6951. Bibcode: 2014ApPhL.104k3902B. http://aip.scitation.org/doi/10.1063/1.4868880.
- ↑ 106.0 106.1 Geissbühler, Jonas; Werner, Jérémie; Martin de Nicolas, Silvia; Barraud, Loris; Hessler-Wyser, Aïcha; Despeisse, Matthieu; Nicolay, Sylvain; Tomasi, Andrea et al. (2015-08-24). "22.5% efficient silicon heterojunction solar cell with molybdenum oxide hole collector" (in en). Applied Physics Letters 107 (8): 081601. doi:10.1063/1.4928747. ISSN 0003-6951. Bibcode: 2015ApPhL.107h1601G. https://www.osti.gov/servlets/purl/1229741.
- ↑ Haase, Felix; Hollemann, Christina; Schäfer, Sören; Merkle, Agnes; Rienäcker, Michael; Krügener, Jan; Brendel, Rolf; Peibst, Robby (November 2018). "Laser contact openings for local poly-Si-metal contacts enabling 26.1%-efficient POLO-IBC solar cells" (in en). Solar Energy Materials and Solar Cells 186: 184–193. doi:10.1016/j.solmat.2018.06.020. https://linkinghub.elsevier.com/retrieve/pii/S0927024818303076.
- ↑ Li, Xingliang; Xu, Qiaojing; Yan, Lingling; Ren, Chengchao; Shi, Biao; Wang, Pengyang; Mazumdar, Sayantan; Hou, Guofu et al. (2020). "Silicon heterojunction-based tandem solar cells: past, status, and future prospects". Nanophotonics 10 (8): 2001–2022. doi:10.1515/nanoph-2021-0034.
- ↑ Ebrahim, Shaker; Soliman, Moataz; Abdel-Fattah, Tarek M. (September 2011). "Hybrid Inorganic–Organic Heterojunction Solar Cell" (in en). Journal of Electronic Materials 40 (9): 2033–2041. doi:10.1007/s11664-011-1671-4. ISSN 0361-5235. Bibcode: 2011JEMat..40.2033E. http://link.springer.com/10.1007/s11664-011-1671-4.
- ↑ Impera, D.; Bivour, M.; Michl, B.; Schubert, M.C.; Hermle, M. (3–5 April 2012). "Characterization of Silicon Heterojunctions on Multicrystalline Absorbers Using Injection-Dependent Photoluminescence Imaging". Proceedings of the 2nd International Conference on Crystalline Silicon Photovoltaics SiliconPV 2012. 27. Leuven, Belgium. pp. 280–286. doi:10.1016/j.egypro.2012.07.064.
- ↑ Green, Martin A (2019-07-16). "Photovoltaic technology and visions for the future". Progress in Energy 1 (1): 013001. doi:10.1088/2516-1083/ab0fa8. ISSN 2516-1083. Bibcode: 2019PrEne...1a3001G. https://iopscience.iop.org/article/10.1088/2516-1083/ab0fa8.
- ↑ Müllerová, J.; Jurečka, S.; Šutta, P. (2006-06-01). "Optical characterization of polysilicon thin films for solar applications" (in en). Solar Energy. SREN ’05 – Solar Renewable Energy News Conference 80 (6): 667–674. doi:10.1016/j.solener.2005.10.009. ISSN 0038-092X. Bibcode: 2006SoEn...80..667M. https://www.sciencedirect.com/science/article/pii/S0038092X05003671.
- ↑ Stuckelberger, Michael; Biron, Rémi; Wyrsch, Nicolas; Haug, Franz-Josef; Ballif, Christophe (September 2017). "Review: Progress in solar cells from hydrogenated amorphous silicon" (in en). Renewable and Sustainable Energy Reviews 76: 1497–1523. doi:10.1016/j.rser.2016.11.190. https://linkinghub.elsevier.com/retrieve/pii/S1364032116309467.
- ↑ Krajangsang, Taweewat; Inthisang, Sorapong; Sritharathikhun, Jaran; Hongsingthong, Aswin; Limmanee, Amornrat; Kittisontirak, Songkiate; Chinnavornrungsee, Perawut; Phatthanakun, Rungrueang et al. (April 2017). "An intrinsic amorphous silicon oxide and amorphous silicon stack passivation layer for crystalline silicon heterojunction solar cells" (in en). Thin Solid Films 628: 107–111. doi:10.1016/j.tsf.2017.03.010. Bibcode: 2017TSF...628..107K. https://linkinghub.elsevier.com/retrieve/pii/S0040609017301852.
- ↑ Tomozeiu, Nicolae (26 September 2011), Predeep, P., ed., "Silicon Oxide (SiOx, 0<x<2): a Challenging Material for Optoelectronics" (in en), Optoelectronics - Materials and Techniques (InTech), doi:10.5772/20156, ISBN 978-953-307-276-0, http://www.intechopen.com/books/optoelectronics-materials-and-techniques/silicon-oxide-siox-0-x-2-a-challenging-material-for-optoelectronics, retrieved 2023-01-27
- ↑ 116.0 116.1 Eperon, Giles E.; Stranks, Samuel D.; Menelaou, Christopher; Johnston, Michael B.; Herz, Laura M.; Snaith, Henry J. (2014). "Formamidinium lead trihalide: a broadly tunable perovskite for efficient planar heterojunction solar cells" (in en). Energy & Environmental Science 7 (3): 982. doi:10.1039/c3ee43822h. ISSN 1754-5692. http://xlink.rsc.org/?DOI=c3ee43822h.
- ↑ Green, Martin A.; Dunlop, Ewan D.; Hohl‐Ebinger, Jochen; Yoshita, Masahiro; Kopidakis, Nikos; Hao, Xiaojing (January 2022). "Solar cell efficiency tables (version 59)" (in en). Progress in Photovoltaics: Research and Applications 30 (1): 3–12. doi:10.1002/pip.3506. ISSN 1062-7995. https://onlinelibrary.wiley.com/doi/10.1002/pip.3506.
- ↑ Bellini, Emiliano (3 May 2023). "Toyota uses Kaneka's 26.63%-efficient solar cells for electric vehicle". pv magazine.
- ↑ Rahman, Tasmiat; To, Alexander; Pollard, Michael E.; Grant, Nicholas E.; Colwell, Jack; Payne, David N.R.; Murphy, John D.; Bagnall, Darren M. et al. (January 2018). "Minimising bulk lifetime degradation during the processing of interdigitated back contact silicon solar cells" (in en). Progress in Photovoltaics: Research and Applications 26 (1): 38–47. doi:10.1002/pip.2928. https://onlinelibrary.wiley.com/doi/10.1002/pip.2928.
- ↑ Staebler, D. L.; Wronski, C. R. (15 August 1977). "Reversible conductivity changes in discharge‐produced amorphous Si" (in en). Applied Physics Letters 31 (4): 292–294. doi:10.1063/1.89674. ISSN 0003-6951. Bibcode: 1977ApPhL..31..292S. http://aip.scitation.org/doi/10.1063/1.89674.
- ↑ Du, Mao-Hua; Zhang, S. B. (2005-11-07). "Topological defects and the Staebler-Wronski effect in hydrogenated amorphous silicon" (in en). Applied Physics Letters 87 (19): 191903. doi:10.1063/1.2130381. ISSN 0003-6951. Bibcode: 2005ApPhL..87s1903D. http://aip.scitation.org/doi/10.1063/1.2130381.
- ↑ Xiangna, Liu; Mingde, Xu (February 1986). "The Staebler-Wronski effect in microcrystalline silicon films". Chinese Physics Letters 3 (2): 73–76. doi:10.1088/0256-307X/3/2/007. ISSN 0256-307X. Bibcode: 1986ChPhL...3...73L. https://iopscience.iop.org/article/10.1088/0256-307X/3/2/007.
- ↑ Biswas, R; Pan, B.C (April 2002). "Defect kinetics in new model of metastability in a-Si:H" (in en). Journal of Non-Crystalline Solids 299–302: 507–510. doi:10.1016/S0022-3093(01)00961-9. Bibcode: 2002JNCS..299..507B. https://linkinghub.elsevier.com/retrieve/pii/S0022309301009619.
- ↑ Stuckelberger, M.; Riesen, Y.; Despeisse, M.; Schüttauf, J.-W.; Haug, F.-J.; Ballif, C. (2014-09-07). "Light-induced Voc increase and decrease in high-efficiency amorphous silicon solar cells" (in en). Journal of Applied Physics 116 (9): 094503. doi:10.1063/1.4894457. ISSN 0021-8979. Bibcode: 2014JAP...116i4503S. http://aip.scitation.org/doi/10.1063/1.4894457.
- ↑ Lord, K.; Yan, B.; Yang, J.; Guha, S. (2001-12-03). "Light-induced increase in the open-circuit voltage of thin-film heterogeneous silicon solar cells". Applied Physics Letters 79 (23): 3800–3802. doi:10.1063/1.1420778. ISSN 0003-6951. Bibcode: 2001ApPhL..79.3800L. https://aip.scitation.org/doi/10.1063/1.1420778.
- ↑ 126.0 126.1 Kobayashi, Eiji; De Wolf, Stefaan; Levrat, Jacques; Christmann, Gabriel; Descoeudres, Antoine; Nicolay, Sylvain; Despeisse, Matthieu; Watabe, Yoshimi et al. (2016-10-10). "Light-induced performance increase of silicon heterojunction solar cells" (in en). Applied Physics Letters 109 (15): 153503. doi:10.1063/1.4964835. ISSN 0003-6951. Bibcode: 2016ApPhL.109o3503K. http://aip.scitation.org/doi/10.1063/1.4964835.
- ↑ Scuto, Andrea; Valenti, Luca; Pierro, Silvio; Foti, Marina; Gerardi, Cosimo; Battaglia, Anna; Lombardo, Salvatore (October 2015). "Role of electric field and electrode material on the improvement of the ageing effects in hydrogenated amorphous silicon solar cells" (in en). Solar Energy Materials and Solar Cells 141: 203–209. doi:10.1016/j.solmat.2015.05.040. https://linkinghub.elsevier.com/retrieve/pii/S0927024815002573.
- ↑ Schüttauf, Jan-Willem A.; van der Werf, Karine H. M.; Kielen, Inge M.; van Sark, Wilfried G. J. H. M.; Rath, Jatindra K.; Schropp, Ruud E. I. (2011-04-11). "Excellent crystalline silicon surface passivation by amorphous silicon irrespective of the technique used for chemical vapor deposition" (in en). Applied Physics Letters 98 (15): 153514. doi:10.1063/1.3579540. ISSN 0003-6951. Bibcode: 2011ApPhL..98o3514S. http://aip.scitation.org/doi/10.1063/1.3579540.
- ↑ Madumelu, Chukwuka; Wright, Brendan; Soeriyadi, Anastasia; Wright, Matthew; Chen, Daniel; Hoex, Bram; Hallam, Brett (December 2020). "Investigation of light-induced degradation in N-Type silicon heterojunction solar cells during illuminated annealing at elevated temperatures" (in en). Solar Energy Materials and Solar Cells 218: 110752. doi:10.1016/j.solmat.2020.110752. https://linkinghub.elsevier.com/retrieve/pii/S0927024820303512.
- ↑ Danel, Adrien; Chaugier, Nicolas; Veirman, Jordi; Varache, Renaud; Albaric, Mickael; Pihan, Etienne (2022-10-21). "Closing the gap between n‐ and p‐type silicon heterojunction solar cells: 24.47% efficiency on lightly doped Ga wafers" (in en). Progress in Photovoltaics: Research and Applications: pip.3635. doi:10.1002/pip.3635. ISSN 1062-7995. https://onlinelibrary.wiley.com/doi/10.1002/pip.3635.
- ↑ Wang, Ethan; Yang, Hsinjin Edwin; Yen, Jerry; Chi, Sunny; Wang, Carl (2013). "Failure Modes Evaluation of PV Module via Materials Degradation Approach" (in en). Energy Procedia 33: 256–264. doi:10.1016/j.egypro.2013.05.066. https://linkinghub.elsevier.com/retrieve/pii/S1876610213000763.
- ↑ 132.0 132.1 Bai, Yu; Zhao, Yilin; Li, Junjun; Chen, Hongyuan; Lambertz, Andreas; Qiu, Qingqing; Qian, Cheng; Shi, Jianhua et al. (18 March 2023). "Lower Levelized Cost of Energy Achievement of Silicon Heterojunction Solar Modules with Low Water Vapor Transmission Rate Encapsulants" (in en). Energy Technology 11 (7). doi:10.1002/ente.202201466. ISSN 2194-4288. https://onlinelibrary.wiley.com/doi/10.1002/ente.202201466.
- ↑ 133.0 133.1 Yamaguchi, Seira; Yamamoto, Chizuko; Ohdaira, Keisuke; Masuda, Atsushi (September 2018). "Comprehensive study of potential-induced degradation in silicon heterojunction photovoltaic cell modules" (in en). Progress in Photovoltaics: Research and Applications 26 (9): 697–708. doi:10.1002/pip.3006. https://onlinelibrary.wiley.com/doi/10.1002/pip.3006.
- ↑ del Cueto, J A; Trudell, D; Sekulic, W (1 November 2005). "Capabilities of the High Voltage Stress Test System at the Outdoor Test Facility". 2005 DOE Solar Energy Technologies Program Review Meeting. Denver, Colorado. https://www.osti.gov/biblio/882606.
- ↑ Harvey, Steven P.; Aguiar, Jeffery A.; Hacke, Peter; Guthrey, Harvey; Johnston, Steve; Al-Jassim, Mowafak (November 2016). "Sodium Accumulation at Potential-Induced Degradation Shunted Areas in Polycrystalline Silicon Modules". IEEE Journal of Photovoltaics 6 (6): 1440–1445. doi:10.1109/JPHOTOV.2016.2601950. ISSN 2156-3381. https://ieeexplore.ieee.org/document/7571190.
- ↑ Pingel, S.; Frank, O.; Winkler, M.; Daryan, S.; Geipel, T.; Hoehne, H.; Berghold, J. (June 2010). "Potential Induced Degradation of solar cells and panels". 2010 35th IEEE Photovoltaic Specialists Conference. pp. 002817–002822. doi:10.1109/PVSC.2010.5616823. ISBN 978-1-4244-5890-5. https://ieeexplore.ieee.org/document/5616823.
- ↑ Hoffmann, Stephan; Koehl, Michael (February 2014). "Effect of humidity and temperature on the potential-induced degradation: Effect of humidity and temperature on the PID" (in en). Progress in Photovoltaics: Research and Applications 22 (2): 173–179. doi:10.1002/pip.2238. https://onlinelibrary.wiley.com/doi/10.1002/pip.2238.
- ↑ Li, Xiaodong; Yang, Yuhao; Jiang, Kai; Huang, Shenglei; Zhao, Wenjie; Li, Zhenfei; Wang, Guangyuan; Han, Anjun et al. (6 April 2023). "Potential‐free sodium‐induced degradation of silicon heterojunction solar cells" (in en). Progress in Photovoltaics: Research and Applications 31 (9): 939–948. doi:10.1002/pip.3698. ISSN 1062-7995. https://onlinelibrary.wiley.com/doi/10.1002/pip.3698.
- ↑ 139.0 139.1 139.2 Hutchins, Mark (20 March 2021). "The weekend read: Bifacial drives PV encapsulant switch". pv magazine (3–2021).
- ↑ Zhu, J.; Montiel-Chicharro, D.; Betts, T.R.; Gottschalg, R. (2017). "Correlation of Degree of EVA Crosslinking with Formation and Discharge of Acetic Acid in PV Modules" (in en). 33rd European Photovoltaic Solar Energy Conference and Exhibition; 1795–1798: 4 pages, 3660 kb. doi:10.4229/EUPVSEC20172017-5DV.3.21. http://www.eupvsec-proceedings.com/proceedings?paper=44217.
- ↑ Masuda, Atsushi; Uchiyama, Naomi; Hara, Yukiko (2015-04-01). "Degradation by acetic acid for crystalline Si photovoltaic modules". Japanese Journal of Applied Physics 54 (4S): 04DR04. doi:10.7567/JJAP.54.04DR04. ISSN 0021-4922. Bibcode: 2015JaJAP..54dDR04M. https://iopscience.iop.org/article/10.7567/JJAP.54.04DR04.
- ↑ 142.0 142.1 Iqbal, Nafis; Chockalingam, Nitin K.; Coleman, Kehley A.; Fina, Jeffrie; Davis, Kristopher O.; Bruckman, Laura S.; Martin, Ina T. (2022-06-05). "Accelerate Cycles of Learning: Unencapsulated Silicon Photovoltaic Cells to Environmental Stressors". 2022 IEEE 49th Photovoltaics Specialists Conference (PVSC). Philadelphia, PA, USA: IEEE. pp. 0668–0674. doi:10.1109/PVSC48317.2022.9938492. ISBN 978-1-7281-6117-4. https://ieeexplore.ieee.org/document/9938492.
- ↑ 143.0 143.1 Karas, Joseph; Sinha, Archana; Buddha, Viswa Sai Pavan; Li, Fang; Moghadam, Farhad; TamizhMani, Govindasamy; Bowden, Stuart; Augusto, Andre (January 2020). "Damp Heat Induced Degradation of Silicon Heterojunction Solar Cells With Cu-Plated Contacts". IEEE Journal of Photovoltaics 10 (1): 153–158. doi:10.1109/JPHOTOV.2019.2941693. ISSN 2156-3381. https://ieeexplore.ieee.org/document/8878168.
- ↑ Sen, Chandany; Wang, Haoran; Wu, Xinyuan; Khan, Muhammad Umair; Chan, Catherine; Abbott, Malcolm; Hoex, Bram (2022). "Four Failure Modes in Silicon Heterojunction Glass-Backsheet Modules" (in en). SSRN Electronic Journal. doi:10.2139/ssrn.4293027. ISSN 1556-5068. https://www.ssrn.com/abstract=4293027.
- ↑ Mittag, M.; Eitner, U.; Neff, T. (2017). "TPedge: Progress on Cost-Efficient and Durable Edge-Sealed PV Modules" (in en). 33rd European Photovoltaic Solar Energy Conference and Exhibition; 48–54: 7 pages, 8203 kb. doi:10.4229/EUPVSEC20172017-1CO.1.4. http://www.eupvsec-proceedings.com/proceedings?paper=40935.
- ↑ Chunduri, Shravan (21 May 2022). "EPE: The Two In One Approach For Module Encapsulation". TaiyangNews. https://taiyangnews.info/technology/the-two-in-one-approach-segment/.
- ↑ Zhao, Derek (6 January 2022). "Post CSPV 2021: Silicon cell, module developments and trends". https://www.infolink-group.com/energy-article/Post-CSPV-2021-Silicon-cell-module-developments-and-trends.
- ↑ Sinha, Archana; Qian, Jiadong; Moffitt, Stephanie L.; Hurst, Katherine; Terwilliger, Kent; Miller, David C.; Schelhas, Laura T.; Hacke, Peter (January 2023). "UV‐induced degradation of high‐efficiency silicon PV modules with different cell architectures" (in en). Progress in Photovoltaics: Research and Applications 31 (1): 36–51. doi:10.1002/pip.3606. ISSN 1062-7995. https://onlinelibrary.wiley.com/doi/10.1002/pip.3606.
- ↑ Yang, Zhengfeng; Li, Yang; Wu, Jiating; Zheng, Yuhe; Fan, Xinyu; Bian, Ting; Masendu, Santana Vimbai; Anton, Romanov et al. (August 2023). "Novel EPE co-extruded encapsulating films with UV down-conversion power gain effect for highly efficient solar cells" (in en). Solar Energy Materials and Solar Cells 257: 112373. doi:10.1016/j.solmat.2023.112373. https://linkinghub.elsevier.com/retrieve/pii/S0927024823001940.
- ↑ Scully, Jules (20 April 2022). "Maxwell, Cybrid use light conversion film to boost HJT module power output". PV-Tech. https://www.pv-tech.org/maxwell-cybrid-use-light-conversion-film-to-boost-hjt-module-power-output/.
- ↑ Xi, Xia (12 November 2022). "异质结技术新"主角"登场!" (in Chinese). PV-Tech. https://www.pv-tech.cn/news/The_new_hero_of_heterostructure_technology_comes_on_the_scene.
![]() | Original source: https://en.wikipedia.org/wiki/Heterojunction solar cell.
Read more |