Chemistry:Unbiunium
Unbiunium | |||||||||||||||||||||
---|---|---|---|---|---|---|---|---|---|---|---|---|---|---|---|---|---|---|---|---|---|
Pronunciation | /ˌuːnbaɪˈuːniəm/ | ||||||||||||||||||||
Alternative names | element 121, eka-actinium | ||||||||||||||||||||
Unbiunium in the periodic table | |||||||||||||||||||||
| |||||||||||||||||||||
Atomic number (Z) | 121 | ||||||||||||||||||||
Group | group n/a (sometimes considered group n/a) | ||||||||||||||||||||
Period | period 8 | ||||||||||||||||||||
Block | g-block (sometimes considered g-block) | ||||||||||||||||||||
Element category | g-block, but probably a superactinide; could be considered a transition metal | ||||||||||||||||||||
Electron configuration | [Og] 8s2 8p1 (predicted)[1] | ||||||||||||||||||||
Electrons per shell | 2, 8, 18, 32, 32, 18, 8, 3 (predicted) | ||||||||||||||||||||
Physical properties | |||||||||||||||||||||
unknown | |||||||||||||||||||||
Phase at STP | unknown | ||||||||||||||||||||
Atomic properties | |||||||||||||||||||||
Oxidation states | (+1), (+3) (predicted)[1][2] | ||||||||||||||||||||
Ionization energies |
| ||||||||||||||||||||
Other properties | |||||||||||||||||||||
CAS Number | 54500-70-8 | ||||||||||||||||||||
History | |||||||||||||||||||||
Naming | IUPAC systematic element name | ||||||||||||||||||||
Main isotopes of unbiunium | |||||||||||||||||||||
| |||||||||||||||||||||
Check temperatures Ubu: no input for C, K, F.
Check temperatures Ubu: no input for C, K, F. Unbiunium, also known as eka-actinium or simply element 121, is the hypothetical chemical element with symbol Ubu and atomic number 121. Unbiunium and Ubu are the temporary systematic IUPAC name and symbol respectively, until a permanent name is decided upon. In the periodic table of the elements, it is expected to be the first of the superactinides, and the third element in the eighth period: analogously to lanthanum and actinium, it could be considered the fifth member of group 3 and the first member of the fifth-row transition metals. It has attracted attention because of some predictions that it may be in the island of stability, although newer calculations expect the island to actually occur at a slightly lower atomic number, closer to copernicium and flerovium.
Unbiunium has not yet been synthesized. Nevertheless, because it is only three elements away from the heaviest known element, oganesson (element 118), its synthesis may come in the near future; it is expected to be one of the last few reachable elements with current technology, and the limit may be anywhere between element 120 and 124. It will also likely be far more difficult to synthesize than the elements known so far up to 118, and still more difficult than elements 119 and 120. The team at RIKEN in Japan has plans to attempt the synthesis of element 121 in the future after its attempts on elements 119 and 120.
The position of unbiunium in the periodic table suggests that it would have similar properties to its lighter congeners, scandium, yttrium, lanthanum, and actinium; however, relativistic effects may cause some of its properties to differ from those expected from a straight application of periodic trends. For example, unbiunium is expected to have a s2p valence electron configuration instead of the s2d of its lighter congeners in group 3, but this is not expected to significantly affect its chemistry, which is predicted to be that of a normal group 3 element; it would on the other hand significantly lower its first ionisation energy beyond what would be expected from periodic trends.
History
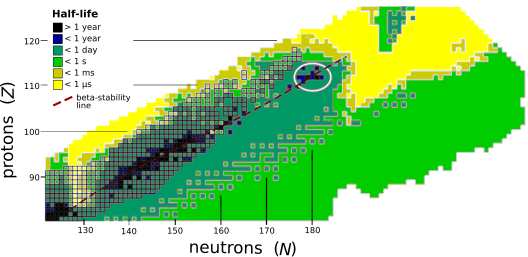
Transactinide elements, such as unbiunium, are produced by nuclear fusion. These fusion reactions can be divided into "hot" and "cold" fusion,[lower-alpha 2] depending on the excitation energy of the compound nucleus produced. In hot fusion reactions, very light, high-energy projectiles are accelerated toward very heavy targets (actinides), giving rise to compound nuclei at high excitation energies (~40–50 MeV) that may fission or evaporate several (3 to 5) neutrons.[5] In cold fusion reactions (which use heavier projectiles, typically from the fourth period, and lighter targets, usually lead and bismuth), the fused nuclei produced have a relatively low excitation energy (~10–20 MeV), which decreases the probability that these products will undergo fission reactions. As the fused nuclei cool to the ground state, they require emission of only one or two neutrons. However, hot fusion reactions tend to produce more neutron-rich products because the actinides have the highest neutron-to-proton ratios of any element that can presently be made in macroscopic quantities; it is currently the only method to produce the superheavy elements from flerovium (element 114) onward.[6]
The stability of nuclei decreases greatly with the increase in atomic number after curium, element 96, whose half-life is four orders of magnitude longer than that of any currently known higher-numbered element. All isotopes with an atomic number above 101 undergo radioactive decay with half-lives of less than 30 hours. No elements with atomic numbers above 82 (after lead) have stable isotopes.[7] Nevertheless, for reasons not yet well understood, there is a slight increase of nuclear stability around atomic numbers 110–114, which leads to the appearance of what is known in nuclear physics as the "island of stability". This concept, proposed by University of California professor Glenn Seaborg and stemming from the stability of the closed nuclear shells around Z = 114 (or possibly 120, 122, 124, or 126) and N = 184, explains why superheavy elements last longer than predicted.[8]
The deformation of nuclei in the superheavy region also slightly alters the magic numbers, so that elements in the "sea of instability" can exist as well, albeit for shorter periods of time. However, the strong force must eventually lose its fight against the Coulomb repulsion of protons: while there might be another brief island of stability around the next closed proton and neutron shells at Z = 164 and N = 318, some proton numbers stranded too far between the first and second islands may not correspond to bound nuclei. Furthermore, the greatest stability is expected to be reached only as one approaches the closed neutron shell at N = 184; the most neutron-rich confirmed nuclides, 293Lv and 294Ts, only reach N = 177.[9][10]
Attempts to synthesize elements 119 and 120 push the limits of current technology, due to the decreasing cross sections of the production reactions and their probably short half-lives,[3] expected to be on the order of microseconds.[1][11] Heavier elements, beginning with element 121, would likely be too short-lived to be detected with current technology, decaying within a microsecond before reaching the detectors.[3] Where this one-microsecond border of half-lives lies is not known, and this may allow the synthesis of elements 121 through 124, with the exact limit depending on the model chosen for predicting nuclide masses. The nuclides 296Uue, 296,299Ubn, 300Ubu, 300,303Ubb, 304Ubt, and 304Ubq, continuing up a possible narrow one-microsecond alpha-decaying corridor, could conceivably be produced in the reactions of projectiles of 50Ti, 54Cr, and 58Fe with targets of 248Cm, 249Bk, and 249Cf.[11]
Previously, important help (characterized as "silver bullets") in the synthesis of superheavy elements came from the deformed nuclear shells around hassium-270 which increased the stability of surrounding nuclei, and the existence of the quasi-stable neutron-rich isotope calcium-48 which could be used as a projectile to produce more neutron-rich isotopes of superheavy elements.[12] (The more neutron-rich a superheavy nuclide is, the closer it is expected to be to the sought-after island of stability.[lower-alpha 3]) Even so, the synthesized isotopes still have fewer neutrons than those expected to be in the island of stability.[15] Furthermore, because of the current impossibility of synthesizing elements beyond californium (Z = 98) in sufficient quantities to create a target, with einsteinium (Z = 99) targets being currently considered, the practical synthesis of elements beyond oganesson requires heavier projectiles, such as titanium-50, chromium-54, iron-58, or nickel-64.[12][16] This however has the drawback of resulting in more symmetrical fusion reactions that are colder and less likely to succeed:[12] the cross-sections for even-numbered elements 122 and beyond with projectiles of 54Cr, 58Fe, and 64Ni quickly become too small to be detected with current equipment, even if the nuclides formed did live long enough to reach the detectors.[17]
Since there is no way to reach N = 184 close to the magic proton shell, the main hope of reaching anywhere near this neutron number in fusion–evaporation reactions is via increasing the proton number past 118, but that also greatly diminishes the cross-sections and may not quite reach N = 184. Using newly available 251Cf targets and intense beams of 48Ca, 50Ti, 54Cr, and 58Fe would allow the production of the more neutron-rich 296Og, 300Ubn, 304Ubb, and 308Ubq, the latter three in the 1n channel that may be indicated by the low neutron binding energy of the compound nucleus 309Ubq* with N = 185 just above the closed shell. 308Ubq would reach the magic neutron number N = 184 and consequently have a higher cross-section for its production.[18] Nevertheless, the growing importance of spontaneous fission as a decay mode over alpha decay in this region of the nuclide chart, as well as the short half-lives, may hamper the production of these nuclides even though they reach the closed neutron shell, and the feasibility of the 1n channel is also questionable.[11] The most plausible way of reaching the center of the island with current technology is to reach nuclides like 299Uue, 295Ts, and 295Lv whose alpha daughters are near enough to the island to undergo electron capture to add the necessary neutrons; nonetheless, the island is expected to be centered around copernicium, element 112, and thus this would not be of much help synthesizing elements beyond 118.[3]
A 2016 calculation of the half-lives of the isotopes of unbiunium from 290Ubu to 339Ubu suggested that those from 290Ubu to 303Ubu would not be bound and would decay through proton emission, those from 304Ubu through 314Ubu would undergo alpha decay, and those from 315Ubu to 339Ubu would undergo spontaneous fission. Only the isotopes from 309Ubu to 314Ubu would have long enough alpha-decay lifetimes to be detected in laboratories, starting decay chains terminating in spontaneous fission at moscovium, tennessine, or ununennium. This would present a grave problem for experiments aiming at synthesizing isotopes of unbiunium if true, because the isotopes whose alpha decay could be observed could not be reached by any presently usable combination of target and projectile.[19] Calculations in 2016 and 2017 by the same authors on elements 123 and 125 suggest a less bleak outcome, with alpha decay chains from the more reachable nuclides 300–307Ubt leading down to bohrium or nihonium; in particular, 304Ubt could be synthesised in the reaction 249Bk(58Fe,3n)304Ubt and would alpha decay through 300Ubu, 296Uue, and 292Ts to the known 288Mc and 284Nh, though the cross-section would likely be extremely low.[20] It has also been suggested that cluster decay might be a significant decay mode in competition with alpha decay and spontaneous fission in the region past Z = 120, which would pose yet another hurdle for experimental identification of these nuclides.[21][22][23]
Synthesis attempts
Past
The synthesis of unbiunium was first attempted in 1977 by bombarding a target of uranium-238 with copper-65 ions at the Gesellschaft für Schwerionenforschung in Darmstadt, Germany :
- 23892U + 6529Cu → 303121Ubu* → no atoms
No atoms were identified.[24]
Planned
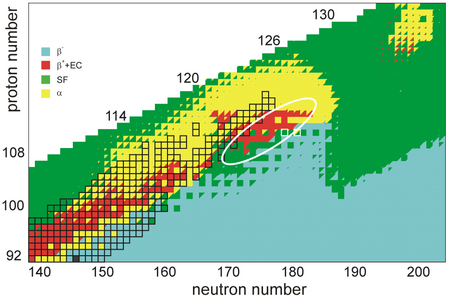
Currently, the beam intensities at superheavy element facilities result in about 1012 projectiles hitting the target per second; this cannot be increased without burning the target and the detector, and producing larger amounts of the increasingly unstable actinides needed for the target is impractical. The team at the Joint Institute for Nuclear Research (JINR) in Dubna is building a new superheavy element factory (SHE-factory) with improved detectors and the ability to work on a smaller scale, but even so continuing beyond element 120 and perhaps 121 would be a great challenge. Krzysztof Rykaczewski, a professor at Oak Ridge National Laboratory which collaborates with Dubna on superheavy elements, has nevertheless predicted that in one generation element 124 would be reached. Chemist, writer, and philosopher of science Eric Scerri has noted that the development of new technologies in the field has been driven by the quest for new elements, so that the inability to proceed beyond elements 120 and 121 with current technologies may not necessarily cause a very long pause in new discoveries.[25]
It is possible that the age of fusion–evaporation reactions to produce new superheavy elements is coming to an end due to the increasingly short half-lives to spontaneous fission and the looming proton drip line, so that new techniques such as nuclear transfer reactions (for example, firing uranium nuclei at each other and letting them exchange protons, potentially producing products with around 120 protons) would be required to reach the superactinides.[25] On the other hand, many changes in techniques have been needed to synthesize transuranium elements, from neutron capture (until Z = 100) to light-ion bombardment (until Z = 110) to cold fusion (until Z = 113) and now hot fusion with 48Ca (until Z = 118), not to mention the essential difference before and after uranium between finding the elements chemically or spectroscopically in nature and synthesizing them.[26] In spite of this, the rate of discovery of new elements has remained at one every two and a half years on average for the past two and a half centuries.[27]
The team at RIKEN has listed the synthesis of element 121 among their future plans after their attempts to synthesize element 120 in 2017–2018 and element 119 in 2019–2020.[28][29] Because the cross-sections of these fusion-evaporation reactions increase with the asymmetry of the reaction, titanium would be a better projectile than chromium for the synthesis of element 121.[30] This necessitates an einsteinium target, since titanium has 22 protons and the remaining 99 must be supplied by the target, and einsteinium is element 99. This poses severe challenges due to the significant heating and damage of the target due to the high radioactivity of einsteinium-254, but it would probably be the most promising approach to element 119 with 48Ca beams and possibly element 121 with 50Ti beams. It would also require working on a smaller scale due to the lower amount of 254Es that can be produced. This small-scale work could in the near future only be carried out in Dubna's SHE-factory.[28]
- 25499Es + 5022Ti → 300121Ubu + 4 10n
- 25499Es + 5022Ti → 301121Ubu + 3 10n
For further elements up to 124, given that increasing the neutron number allows for getting closer to the predicted closed neutron shell at N = 184 that would confer stability, the use of weakly radioactive iron-60 instead of stable iron-58 as a projectile has been considered. 60Fe has a half-life of 2.6 million years.[28] The isotopes 299Ubu, 300Ubu, and 301Ubu that could be produced in these reactions via the 3n and 4n channels are expected to be the only reachable unbiunium isotopes with half-lives long enough for detection; the cross-sections would nevertheless push the limits of what can currently be detected. They would decay through isotopes of ununennium that could be produced by cross-bombardments in the 248Cm+51V or 249Bk+50Ti reactions, which will be tried at RIKEN and JINR respectively in 2019–2020, down through known isotopes of tennessine and moscovium synthesized in the 249Bk+48Ca and 243Am+48Ca reactions.[3] The multiplicity of excited states populated by the alpha decay of odd nuclei may however preclude clear cross-bombardment cases, as was seen in the controversial link between 293Ts and 289Mc.[31][32] Heavier isotopes are expected to be more stable; 320Ubu is predicted to be the most stable unbiunium isotope, but there is no way to synthesize it with current technology as no combination of usable target and projectile could provide enough neutrons.[2]
The laboratories at RIKEN in Japan and at the JINR in Russia are best suited to these experiments as they are the only ones in the world where long beam times are accessible for reactions with such low predicted cross-sections.[33]
Naming
Using Mendeleev's nomenclature for unnamed and undiscovered elements, unbiunium should be known as eka-actinium. Using the 1979 IUPAC recommendations, the element should be temporarily called unbiunium (symbol Ubu) until it is discovered, the discovery is confirmed, and a permanent name chosen.[34] Although widely used in the chemical community on all levels, from chemistry classrooms to advanced textbooks, the recommendations are mostly ignored among scientists who work theoretically or experimentally on superheavy elements, who call it "element 121", with the symbol E121, (121), or 121.[1]
Predicted chemistry
Unbiunium is predicted to be the first element of an unprecedentedly long transition series, called the superactinides in analogy to the earlier actinides. While its behavior is not likely to be very distinct from its lighter congeners in group 3,[1] it is likely to pose a limit to the applicability of the periodic law; after element 121, the 5g, 6f, 7d, and 8p1/2 orbitals are expected to fill up together due to their very close energies, and around the elements in the late 150s and 160s the 9s, 9p1/2, and 8p3/2 subshells join in, so that the chemistry of the elements just beyond 121 and 122 (the last for which complete calculations have been conducted) is expected to be so similar that their position in the periodic table would be purely a formal matter.[35] Like its lighter congeners, unbiunium could be considered to begin the 7d series of elements, which would be the fifth row of the transition metals.[1]
Based on the Aufbau principle, one would expect the 5g subshell to begin filling at unbiunium. However, the f-block is theoretically expected to begin at lanthanum and actinium, but neither element shows significant f-orbital involvement. It is predicted that a similar situation of delayed "radial" collapse might happen for unbiunium, so that the 5g orbitals do not start filling until around element 125. This is similar to the situation with lanthanum, where the 4f shell has not yet undergone the sudden contraction and lowering in energy to the level it will have in the lanthanide series; even in the next element, cerium, this contraction is not yet complete enough to avoid occupation of the 5d orbital.[36] The delay in the contraction of the 5g orbitals is expected to be so large that occupation of the 6f, 7d, and 8p1/2 subshells cannot be avoided in any superactinide.[1] Because of the lack of radial nodes in the 5g orbitals, analogous to the 4f but not the 5f orbitals, the position of unbiunium in the periodic table is expected to be more akin to that of lanthanum than that of actinium among its congeners, and some have proposed to rename the superactinides as "superlanthanides" for that reason.[37] The lack of radial nodes in the 4f orbitals contribute to their core-like behavior in the lanthanide series, unlike the more valence-like 5f orbitals in the actinides; however, the relativistic expansion and destabilization of the 5g orbitals should partially compensate for their lack of radial nodes and hence smaller extent.[38]
While the lighter group 3 elements fill d-orbitals (Sc, [Ar]3d14s2; Y, [Kr]4d15s2; La, [Xe]5d16s2; Ac, [Rn]6d17s2), unbiunium is expected to fill the 8p1/2 orbital instead due to its relativistic stabilization, with a configuration of [Og]8s28p1. Nevertheless, the expected [Og]7d18s2 configuration is expected to be a low-lying excited state at only 0.412 eV.[39] The electron configurations of the ions of unbiunium are expected to be Ubu+, [Og]8s2; Ubu2+, [Og]8s1; and Ubu3+, [Og].[40] The 8p electron of unbiunium is expected to be very loosely bound, so that its predicted ionization energy of 4.45 eV is lower than that of ununennium (4.53 eV) and all known elements except for the alkali metals from potassium downwards. A similar dramatic reduction in ionization energy is also seen in lawrencium, another element having an anomalous s2p configuration instead of the expected ds2 due to relativistic effects.[1]
Despite the change in electron configuration, unbiunium is not expected to behave chemically very differently from its lighter congeners. A 2016 calculation on unbiunium monofluoride (UbuF) showed similarities between the valence orbitals of unbiunium in this molecule and those of actinium in actinium monofluoride (AcF); in both molecules, the highest occupied molecular orbital is expected to be non-bonding, unlike in the superficially more similar nihonium monofluoride (NhF) where it is bonding. Nihonium has the electron configuration [Rn]5f146d107s27p1, with an s2p valence configuration. Unbiunium may hence be somewhat like lawrencium in having an anomalous s2p configuration from relativistic effects that does not affect its chemistry: the bond dissociation energies, bond lengths, and polarizabilities of the UbuF molecule are expected to continue the trend down group 3. Hence lawrencium and unbiunium are expected to be similar to lutetium and actinium respectively, and not thallium and nihonium as would be expected from looking at their electron configurations. The Ubu–F bond is expected to be strong and polarized, just like for the lighter group 3 monofluorides.[2]
Analogous to its lighter congeners, the non-bonding electrons on unbiunium in UbuF are expected to be able to bond to extra atoms or groups, resulting in the formation of the unbiunium trihalides UbuX3, analogous to ScX3, YX3, LaX3, and AcX3. Hence, the main oxidation state of unbiunium in its compounds should be +3, although the closeness of the valence subshells' energy levels may permit higher oxidation states, just like in elements 119 and 120.[1][2][37] The standard electrode potential for the Ubu3+/Ubu couple is predicted as −2.1 V.[1]
Notes
- ↑ Similarly to the controversy about group 3, it is not certain if actinium is truly the lighter congener of unbiunium.
- ↑ Despite the name, "cold fusion" in the context of superheavy element synthesis is a distinct concept from the idea that nuclear fusion can be achieved in room temperature conditions (see cold fusion).[4]
- ↑ Stable isotopes of the lightest elements usually have a neutron–proton ratio close or equal to one (for example, the only stable isotope of aluminium has 13 protons and 14 neutrons,[13] making a neutron–proton ratio of 1.077). However, isotopes of heavier elements have higher neutron–proton ratios, increasing with the number of protons (iodine's only stable isotope has 53 protons and 74 neutrons, neutron–proton ratio of 1.396; gold's only stable isotope has 79 protons and 118 neutrons, neutron–proton ratio of 1.494; plutonium's most stable isotope has 94 protons and 150 neutrons, neutron–proton ratio of 1.596).[13] The trend is expected to continue to the superheavy elements,[14] making it difficult to synthesize their most stable isotopes, because the neutron–proton ratios of the elements they are synthesized from are lower than the expected ratios of the most stable isotopes of the superheavy elements.
References
- ↑ 1.00 1.01 1.02 1.03 1.04 1.05 1.06 1.07 1.08 1.09 1.10 Hoffman, Darleane C.; Lee, Diana M.; Pershina, Valeria (2006). "Transactinides and the future elements". in Morss; Edelstein, Norman M.; Fuger, Jean. The Chemistry of the Actinide and Transactinide Elements (3rd ed.). Dordrecht, The Netherlands: Springer Science+Business Media. ISBN 978-1-4020-3555-5.
- ↑ 2.0 2.1 2.2 2.3 Amador, Davi H. T.; de Oliveira, Heibbe C. B.; Sambrano, Julio R.; Gargano, Ricardo; de Macedo, Luiz Guilherme M. (12 September 2016). "4-Component correlated all-electron study on Eka-actinium Fluoride (E121F) including Gaunt interaction: Accurate analytical form, bonding and influence on rovibrational spectra". Chemical Physics Letters 662: 169–175. doi:10.1016/j.cplett.2016.09.025. Bibcode: 2016CPL...662..169A.
- ↑ 3.0 3.1 3.2 3.3 3.4 Zagrebaev, Valeriy; Karpov, Alexander; Greiner, Walter (2013). "Future of superheavy element research: Which nuclei could be synthesized within the next few years?". Journal of Physics (IOP Publishing Ltd) 420: 012001. doi:10.1088/1742-6596/420/1/012001. http://nrv.jinr.ru/pdf_file/J_phys_2013.pdf.
- ↑ Fleischmann, Martin; Pons, Stanley (1989). "Electrochemically induced nuclear fusion of deuterium". Journal of Electroanalytical Chemistry and Interfacial Electrochemistry 261 (2): 301–308. doi:10.1016/0022-0728(89)80006-3.
- ↑ Barber, Robert C.; Gäggeler, Heinz W.; Karol, Paul J.; Nakahara, Hiromichi; Vardaci, Emanuele; Vogt, Erich (2009). "Discovery of the element with atomic number 112 (IUPAC Technical Report)". Pure and Applied Chemistry 81 (7): 1331. doi:10.1351/PAC-REP-08-03-05.
- ↑ Armbruster, Peter; Munzenberg, Gottfried (1989). "Creating superheavy elements". Scientific American 34: 36–42.
- ↑ de Marcillac, Pierre; Coron, Noël; Dambier, Gérard; Leblanc, Jacques; Moalic, Jean-Pierre (2003). "Experimental detection of α-particles from the radioactive decay of natural bismuth". Nature 422 (6934): 876–878. doi:10.1038/nature01541. PMID 12712201. Bibcode: 2003Natur.422..876D.
- ↑ Considine, Glenn D.; Kulik, Peter H. (2002). Van Nostrand's scientific encyclopedia (9th ed.). Wiley-Interscience. ISBN 978-0-471-33230-5. OCLC 223349096.
- ↑ 9.0 9.1 Greiner, Walter (2013). "Nuclei: superheavy–superneutronic–strange–and of antimatter". Journal of Physics: Conference Series 413: 012002. doi:10.1088/1742-6596/413/1/012002. http://inspirehep.net/record/1221632/files/jpconf13_413_012002.pdf. Retrieved 30 April 2017.
- ↑ Kaji, Daiya; Morita, Kosuke; Morimoto, Kouji; Haba, Hiromitsu; Asai, Masato; Fujita, Kunihiro; Gan, Zaiguo; Geissel, Hans et al. (2017). "Study of the Reaction 48Ca + 248Cm → 296Lv* at RIKEN-GARIS". Journal of the Physical Society of Japan 86 (3): 034201–1–7. doi:10.7566/JPSJ.86.034201. Bibcode: 2017JPSJ...86c4201K.
- ↑ 11.0 11.1 11.2 11.3 Karpov, Alexander; Zagrebaev, Valery; Greiner, Walter (1 April 2015). "Superheavy Nuclei: which regions of nuclear map are accessible in the nearest studies". Texas A & M University. http://cyclotron.tamu.edu/she2015/assets/pdfs/presentations/Karpov_SHE_2015_TAMU.pdf.
- ↑ 12.0 12.1 12.2 Folden III, C. M.; Mayorov, D. A.; Werke, T. A.; Alfonso, M. C.; Bennett, M. E.; DeVanzo, M. J. (2013). "Prospects for the discovery of the next new element: Influence of projectiles with Z > 20". Journal of Physics: Conference Series (IOP Publishing Ltd.) 420 (1): 012007. doi:10.1088/1742-6596/420/1/012007.
- ↑ 13.0 13.1 Audi, Georges; Bersillon, Olivier; Blachot, Jean et al. (2003). "The NUBASE evaluation of nuclear and decay properties". Nuclear Physics A 729 (1): 3–128. doi:10.1016/j.nuclphysa.2003.11.001. Bibcode: 2003NuPhA.729....3A. Archived from the original on 2011-07-20. https://web.archive.org/web/20110720233206/http://amdc.in2p3.fr/nubase/Nubase2003.pdf.
- ↑ Karpov, A. V.; Zagrebaev, V. I.; Palenzuela, Y. Martinez; Greiner, Walter (2013). "Superheavy Nuclei: Decay and Stability". Exciting Interdisciplinary Physics. p. 69. doi:10.1007/978-3-319-00047-3_6. ISBN 978-3-319-00046-6.
- ↑ "Universal nuclide chart". Nucleonica. Institute for Transuranium Elements. 2007–2012. http://www.nucleonica.net/unc.aspx. Retrieved 2012-07-03. (registration required)
- ↑ Gan, ZaiGuo; Zhou, XiaoHong; Huang, MingHui; Feng, ZhaoQing; Li, JunQing (August 2011). "Predictions of synthesizing element 119 and 120". Science China Physics, Mechanics and Astronomy (Springer) 54 (1): 61–66. doi:10.1007/s11433-011-4436-4. Bibcode: 2011SCPMA..54...61G.
- ↑ Adamian, G. G.; Antonenko, N. V.; Bezbakh, A. N.; Sargsyan, V. V.; Scheid, W.; Shneidman, T. M. (1 April 2015). "Isotopic trends in the production of superheavy nuclei". Texas A & M University. http://cyclotron.tamu.edu/she2015/assets/pdfs/presentations/Adamian_SHE_2015_TAMU.pdf.
- ↑ Rykaczewski, Krzysztof P. (July 2016). "Super Heavy Elements and Nuclei". MSU. https://people.nscl.msu.edu/~iwasaki/EBSS2016/KR_EBSS2016.pdf.
- ↑ Santhosh, K. P.; Nithya, C. (27 September 2016). "Predictions on the alpha decay chains of superheavy nuclei with Z = 121 within the range 290 ≤ A ≤ 339". International Journal of Modern Physics E 25 (10): 1650079. doi:10.1142/S0218301316500798. Bibcode: 2016IJMPE..2550079S. https://arxiv.org/ftp/arxiv/papers/1609/1609.05495.pdf. Retrieved 30 April 2017.
- ↑ Santhosh, K. P.; Nithya, C. (28 December 2016). "Theoretical predictions on the decay properties of superheavy nuclei Z = 123 in the region 297 ≤ A ≤ 307". The European Physical Journal A 52 (371). doi:10.1140/epja/i2016-16371-y. Bibcode: 2016EPJA...52..371S.
- ↑ Santhosh, K. P.; Sukumaran, Indu (25 January 2017). "Decay of heavy particles from Z = 125 superheavy nuclei in the region A = 295–325 using different versions of proximity potential". International Journal of Modern Physics E 26 (3): 1750003. doi:10.1142/S0218301317500033. Bibcode: 2017IJMPE..2650003S.
- ↑ Poenaru, Dorin N.; Gherghescu, R. A.; Greiner, W.; Shakib, Nafiseh (September 2014). "How Rare Is Cluster Decay of Superheavy Nuclei?". Nuclear Physics: Present and Future FIAS Interdisciplinary Science Series 2015. doi:10.1007/978-3-319-10199-6_13.
- ↑ Poenaru, Dorin N.; Gherghescu, R. A.; Greiner, W. (March 2012). "Cluster decay of superheavy nuclei". Physical Review C 85 (3). doi:10.1103/PhysRevC.85.034615. Bibcode: 2012PhRvC..85c4615P. https://www.researchgate.net/publication/235507943_Cluster_decay_of_superheavy_nuclei. Retrieved 2 May 2017.
- ↑ Hofmann, Sigurd (2002). On Beyond Uranium. Taylor & Francis. p. 105. ISBN 0-415-28496-1.
- ↑ 25.0 25.1 Krämer, Katrina (29 January 2016). "Beyond element 118: the next row of the periodic table". Chemistry World. https://www.chemistryworld.com/news/beyond-element-118-the-next-row-of-the-periodic-table/9400.article.
- ↑ Oganessian, Yu. Ts. (27 January 2017). "Discovering Superheavy Elements". Oak Ridge National Laboratory. https://www.youtube.com/watch?v=F1sCiP72SY4. Retrieved 21 April 2017.
- ↑ Karol, Paul J. (1 January 2017). "The Periodic Table (continued?): Eka-francium Et Seq.". Chemistry International 39 (1): 10–14. doi:10.1515/ci-2017-0104. https://www.degruyter.com/view/j/ci.2017.39.issue-1/ci-2017-0104/ci-2017-0104.xml. Retrieved 1 May 2017.
- ↑ 28.0 28.1 28.2 Roberto, J. B. (31 March 2015). "Actinide Targets for Super-Heavy Element Research". Texas A & M University. http://cyclotron.tamu.edu/she2015/assets/pdfs/presentations/Roberto_SHE_2015_TAMU.pdf.
- ↑ Morita, Kōsuke (5 February 2016). "The Discovery of Element 113". https://www.youtube.com/watch?v=kGVkkVMgvOg.
- ↑ Siwek-Wilczyńska, K.; Cap, T.; Wilczyński, J. (April 2010). "How can one synthesize the element Z = 120?". International Journal of Modern Physics E 19 (4): 500. doi:10.1142/S021830131001490X. Bibcode: 2010IJMPE..19..500S.
- ↑ Forsberg, U.; Rudolph, D.; Fahlander, C.; Golubev, P.; Sarmiento, L. G.; Åberg, S.; Block, M.; Düllmann, Ch. E. et al. (9 July 2016). "A new assessment of the alleged link between element 115 and element 117 decay chains". Physics Letters B 760 (2016): 293–6. doi:10.1016/j.physletb.2016.07.008. Bibcode: 2016PhLB..760..293F. http://portal.research.lu.se/portal/files/9762047/PhysLettB760_293_2016.pdf. Retrieved 2 April 2016.
- ↑ Forsberg, Ulrika; Fahlander, Claes; Rudolph, Dirk (2016). "Congruence of decay chains of elements 113, 115, and 117". Nobel Symposium NS160 – Chemistry and Physics of Heavy and Superheavy Elements. doi:10.1051/epjconf/201613102003. http://www.epj-conferences.org/articles/epjconf/pdf/2016/26/epjconf-NS160-02003.pdf.
- ↑ Hagino, Kouichi; Hofmann, Sigurd; Miyatake, Hiroari; Nakahara, Hiromichi (2012). "平成23年度 研究業績レビュー(中間レビュー)の実施について". RIKEN. http://www.riken.jp/~/media/riken/about/reports/evaluation/rnc/rep/rnc-morita2012-report-e.pdf.
- ↑ Chatt, J. (1979). "Recommendations for the naming of elements of atomic numbers greater than 100". Pure and Applied Chemistry 51 (2): 381–384. doi:10.1351/pac197951020381.
- ↑ Loveland, Walter (2015). "The Quest for Superheavy Elements". 2015 National Nuclear Physics Summer School. http://www.int.washington.edu/NNPSS/2015/nnpss2015_SHE_Loveland.pdf.
- ↑ Greenwood, Norman N.; Earnshaw, Alan (1997). Chemistry of the Elements (2nd ed.). Butterworth-Heinemann. ISBN 978-0-08-037941-8.
- ↑ 37.0 37.1 Pyykkö, Pekka (2011). "A suggested periodic table up to Z≤ 172, based on Dirac–Fock calculations on atoms and ions". Physical Chemistry Chemical Physics 13 (1): 161–8. doi:10.1039/c0cp01575j. PMID 20967377. Bibcode: 2011PCCP...13..161P.
- ↑ Kaupp, Martin (1 December 2006). "The role of radial nodes of atomic orbitals for chemical bonding and the periodic table". Journal of Computational Chemistry 28 (1): 320–5. doi:10.1002/jcc.20522. PMID 17143872. http://depa.fquim.unam.mx/amyd/archivero/LecturasobreNodosRadiales_12854.pdf. Retrieved 14 October 2016.
- ↑ Eliav, Ephraim; Shmulyian, Sergei; Kaldor, Uzi; Ishikawa, Yasuyuki (1998). "Transition energies of lanthanum, actinium, and eka-actinium (element 121)". The Journal of Chemical Physics 109 (10): 3954. doi:10.1063/1.476995. Bibcode: 1998JChPh.109.3954E.
- ↑ Dolg, Michael (2015). Computational Methods in Lanthanide and Actinide Chemistry. John Wiley & Sons. p. 35. ISBN 978-1-118-68829-8.
Further reading
- Kaldor, U. (2005). "Superheavy Elements—Chemistry and Spectroscopy". Encyclopedia of Computational Chemistry. doi:10.1002/0470845015.cu0044. ISBN 0-470-84501-5.
- Seaborg, G. T. (1968). "Elements Beyond 100, Present Status and Future Prospects". Annual Review of Nuclear Science 18: 53–15. doi:10.1146/annurev.ns.18.120168.000413. Bibcode: 1968ARNPS..18...53S.