Biology:Epigenetics
In biology, epigenetics is the study of heritable traits, or a stable change of cell function, that happen without changes to the DNA sequence.[1] The Greek prefix epi- (ἐπι- "over, outside of, around") in epigenetics implies features that are "on top of" or "in addition to" the traditional (DNA sequence based) genetic mechanism of inheritance.[2] Epigenetics usually involves a change that is not erased by cell division, and affects the regulation of gene expression.[3] Such effects on cellular and physiological phenotypic traits may result from environmental factors, or be part of normal development. They can lead to cancer.[4]
The term also refers to the mechanism of changes: functionally relevant alterations to the genome that do not involve mutation of the nucleotide sequence. Examples of mechanisms that produce such changes are DNA methylation and histone modification, each of which alters how genes are expressed without altering the underlying DNA sequence.[5] Further, non-coding RNA sequences have shown to play a key role in the regulation of gene expression.[6] Gene expression can be controlled through the action of repressor proteins that attach to silencer regions of the DNA. These epigenetic changes may last through cell divisions for the duration of the cell's life, and may also last for multiple generations, even though they do not involve changes in the underlying DNA sequence of the organism;[7] instead, non-genetic factors cause the organism's genes to behave (or "express themselves") differently.[8]
One example of an epigenetic change in eukaryotic biology is the process of cellular differentiation. During morphogenesis, totipotent stem cells become the various pluripotent cell lines of the embryo, which in turn become fully differentiated cells. In other words, as a single fertilized egg cell – the zygote – continues to divide, the resulting daughter cells change into all the different cell types in an organism, including neurons, muscle cells, epithelium, endothelium of blood vessels, etc., by activating some genes while inhibiting the expression of others.[9]
Definitions
The term epigenesis has a generic meaning of "extra growth" that has been used in English since the 17th century.[10] In scientific publications, the term epigenetics started to appear in the 1930s (see Fig. on the right). However, its contemporary meaning emerged only in the 1990s.[11]
A definition of the concept of epigenetic trait as a "stably heritable phenotype resulting from changes in a chromosome without alterations in the DNA sequence" was formulated at a Cold Spring Harbor meeting in 2008,[12] although alternate definitions that include non-heritable traits are still being used widely.[13]
Waddington's canalisation, 1940s
The hypothesis of epigenetic changes affecting the expression of chromosomes was put forth by the Russian biologist Nikolai Koltsov.[14] From the generic meaning, and the associated adjective epigenetic, British embryologist C. H. Waddington coined the term epigenetics in 1942 as pertaining to epigenesis, in parallel to Valentin Haecker's 'phenogenetics' (Phänogenetik).[15] Epigenesis in the context of the biology of that period referred to the differentiation of cells from their initial totipotent state during embryonic development.[16]
When Waddington coined the term, the physical nature of genes and their role in heredity was not known. He used it instead as a conceptual model of how genetic components might interact with their surroundings to produce a phenotype; he used the phrase "epigenetic landscape" as a metaphor for biological development. Waddington held that cell fates were established during development in a process he called canalisation much as a marble rolls down to the point of lowest local elevation.[17] Waddington suggested visualising increasing irreversibility of cell type differentiation as ridges rising between the valleys where the marbles (analogous to cells) are travelling.[18]
In recent times, Waddington's notion of the epigenetic landscape has been rigorously formalized in the context of the systems dynamics state approach to the study of cell-fate.[19][20] Cell-fate determination is predicted to exhibit certain dynamics, such as attractor-convergence (the attractor can be an equilibrium point, limit cycle or strange attractor) or oscillatory.[20]
Contemporary
Robin Holliday defined in 1990 epigenetics as "the study of the mechanisms of temporal and spatial control of gene activity during the development of complex organisms."[21]
More recent usage of the word in biology follows stricter definitions. As defined by Arthur Riggs and colleagues, it is "the study of mitotically and/or meiotically heritable changes in gene function that cannot be explained by changes in DNA sequence."[22]
The term has also been used, however, to describe processes which have not been demonstrated to be heritable, such as some forms of histone modification. Consequently, there are attempts to redefine "epigenetics" in broader terms that would avoid the constraints of requiring heritability. For example, Adrian Bird defined epigenetics as "the structural adaptation of chromosomal regions so as to register, signal or perpetuate altered activity states."[7] This definition would be inclusive of transient modifications associated with DNA repair or cell-cycle phases as well as stable changes maintained across multiple cell generations, but exclude others such as templating of membrane architecture and prions unless they impinge on chromosome function. Such redefinitions however are not universally accepted and are still subject to debate.[23] The NIH "Roadmap Epigenomics Project", which ran from 2008 to 2017, uses the following definition: "For purposes of this program, epigenetics refers to both heritable changes in gene activity and expression (in the progeny of cells or of individuals) and also stable, long-term alterations in the transcriptional potential of a cell that are not necessarily heritable."[24] In 2008, a consensus definition of the epigenetic trait, a "stably heritable phenotype resulting from changes in a chromosome without alterations in the DNA sequence," was made at a Cold Spring Harbor meeting.[12]
The similarity of the word to "genetics" has generated many parallel usages. The "epigenome" is a parallel to the word "genome", referring to the overall epigenetic state of a cell, and epigenomics refers to global analyses of epigenetic changes across the entire genome.[13] The phrase "genetic code" has also been adapted – the "epigenetic code" has been used to describe the set of epigenetic features that create different phenotypes in different cells from the same underlying DNA sequence. Taken to its extreme, the "epigenetic code" could represent the total state of the cell, with the position of each molecule accounted for in an epigenomic map, a diagrammatic representation of the gene expression, DNA methylation and histone modification status of a particular genomic region. More typically, the term is used in reference to systematic efforts to measure specific, relevant forms of epigenetic information such as the histone code or DNA methylation patterns.[citation needed]
Mechanisms
Covalent modification of either DNA (e.g. cytosine methylation and hydroxymethylation) or of histone proteins (e.g. lysine acetylation, lysine and arginine methylation, serine and threonine phosphorylation, and lysine ubiquitination and sumoylation) play central roles in many types of epigenetic inheritance. Therefore, the word "epigenetics" is sometimes used as a synonym for these processes. However, this can be misleading. Chromatin remodeling is not always inherited, and not all epigenetic inheritance involves chromatin remodeling.[25] In 2019, a further lysine modification appeared in the scientific literature linking epigenetics modification to cell metabolism, i.e. Lactylation[26]
Because the phenotype of a cell or individual is affected by which of its genes are transcribed, heritable transcription states can give rise to epigenetic effects. There are several layers of regulation of gene expression. One way that genes are regulated is through the remodeling of chromatin. Chromatin is the complex of DNA and the histone proteins with which it associates. If the way that DNA is wrapped around the histones changes, gene expression can change as well. Chromatin remodeling is accomplished through two main mechanisms:
- The first way is post translational modification of the amino acids that make up histone proteins. Histone proteins are made up of long chains of amino acids. If the amino acids that are in the chain are changed, the shape of the histone might be modified. DNA is not completely unwound during replication. It is possible, then, that the modified histones may be carried into each new copy of the DNA. Once there, these histones may act as templates, initiating the surrounding new histones to be shaped in the new manner. By altering the shape of the histones around them, these modified histones would ensure that a lineage-specific transcription program is maintained after cell division.
- The second way is the addition of methyl groups to the DNA, mostly at CpG sites, to convert cytosine to 5-methylcytosine. 5-Methylcytosine performs much like a regular cytosine, pairing with a guanine in double-stranded DNA. However, when methylated cytosines are present in CpG sites in the promoter and enhancer regions of genes, the genes are often repressed.[27][28] When methylated cytosines are present in CpG sites in the gene body (in the coding region excluding the transcription start site) expression of the gene is often enhanced. Transcription of a gene usually depends on a transcription factor binding to a (10 base or less) recognition sequence at the enhancer that interacts with the promoter region of that gene (Gene expression).[29] About 22% of transcription factors are inhibited from binding when the recognition sequence has a methylated cytosine. In addition, presence of methylated cytosines at a promoter region can attract methyl-CpG-binding domain (MBD) proteins. All MBDs interact with nucleosome remodeling and histone deacetylase complexes, which leads to gene silencing. In addition, another covalent modification involving methylated cytosine is its demethylation by TET enzymes. Hundreds of such demethylations occur, for instance, during learning and memory forming events in neurons.[30][31]
There is frequently a reciprocal relationship between DNA methylation and histone lysine methylation.[32] For instance, the methyl binding domain protein MBD1, attracted to and associating with methylated cytosine in a DNA CpG site, can also associate with H3K9 methyltransferase activity to methylate histone 3 at lysine 9. On the other hand, DNA maintenance methylation by DNMT1 appears to partly rely on recognition of histone methylation on the nucleosome present at the DNA site to carry out cytosine methylation on newly synthesized DNA.[32] There is further crosstalk between DNA methylation carried out by DNMT3A and DNMT3B and histone methylation so that there is a correlation between the genome-wide distribution of DNA methylation and histone methylation.[33]
Mechanisms of heritability of histone state are not well understood; however, much is known about the mechanism of heritability of DNA methylation state during cell division and differentiation. Heritability of methylation state depends on certain enzymes (such as DNMT1) that have a higher affinity for 5-methylcytosine than for cytosine. If this enzyme reaches a "hemimethylated" portion of DNA (where 5-methylcytosine is in only one of the two DNA strands) the enzyme will methylate the other half. However, it is now known that DNMT1 physically interacts with the protein UHRF1. UHRF1 has been recently recognized as essential for DNMT1-mediated maintenance of DNA methylation. UHRF1 is the protein that specifically recognizes hemi-methylated DNA, therefore bringing DNMT1 to its substrate to maintain DNA methylation.[33]
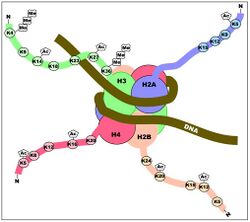
Although histone modifications occur throughout the entire sequence, the unstructured N-termini of histones (called histone tails) are particularly highly modified. These modifications include acetylation, methylation, ubiquitylation, phosphorylation, sumoylation, ribosylation and citrullination. Acetylation is the most highly studied of these modifications. For example, acetylation of the K14 and K9 lysines of the tail of histone H3 by histone acetyltransferase enzymes (HATs) is generally related to transcriptional competence[35] (see Figure).
One mode of thinking is that this tendency of acetylation to be associated with "active" transcription is biophysical in nature. Because it normally has a positively charged nitrogen at its end, lysine can bind the negatively charged phosphates of the DNA backbone. The acetylation event converts the positively charged amine group on the side chain into a neutral amide linkage. This removes the positive charge, thus loosening the DNA from the histone. When this occurs, complexes like SWI/SNF and other transcriptional factors can bind to the DNA and allow transcription to occur. This is the "cis" model of the epigenetic function. In other words, changes to the histone tails have a direct effect on the DNA itself. [36]
Another model of epigenetic function is the "trans" model. In this model, changes to the histone tails act indirectly on the DNA. For example, lysine acetylation may create a binding site for chromatin-modifying enzymes (or transcription machinery as well). This chromatin remodeler can then cause changes to the state of the chromatin. Indeed, a bromodomain – a protein domain that specifically binds acetyl-lysine – is found in many enzymes that help activate transcription, including the SWI/SNF complex. It may be that acetylation acts in this and the previous way to aid in transcriptional activation.
The idea that modifications act as docking modules for related factors is borne out by histone methylation as well. Methylation of lysine 9 of histone H3 has long been associated with constitutively transcriptionally silent chromatin (constitutive heterochromatin) (see bottom Figure). It has been determined that a chromodomain (a domain that specifically binds methyl-lysine) in the transcriptionally repressive protein HP1 recruits HP1 to K9 methylated regions. One example that seems to refute this biophysical model for methylation is that tri-methylation of histone H3 at lysine 4 is strongly associated with (and required for full) transcriptional activation (see top Figure). Tri-methylation, in this case, would introduce a fixed positive charge on the tail.
It has been shown that the histone lysine methyltransferase (KMT) is responsible for this methylation activity in the pattern of histones H3 & H4. This enzyme utilizes a catalytically active site called the SET domain (Suppressor of variegation, Enhancer of Zeste, Trithorax). The SET domain is a 130-amino acid sequence involved in modulating gene activities. This domain has been demonstrated to bind to the histone tail and causes the methylation of the histone.[37]
Differing histone modifications are likely to function in differing ways; acetylation at one position is likely to function differently from acetylation at another position. Also, multiple modifications may occur at the same time, and these modifications may work together to change the behavior of the nucleosome. The idea that multiple dynamic modifications regulate gene transcription in a systematic and reproducible way is called the histone code, although the idea that histone state can be read linearly as a digital information carrier has been largely debunked. One of the best-understood systems that orchestrate chromatin-based silencing is the SIR protein based silencing of the yeast hidden mating-type loci HML and HMR.
DNA methylation
DNA methylation frequently occurs in repeated sequences, and helps to suppress the expression and mobility of 'transposable elements':[38] Because 5-methylcytosine can be spontaneously deaminated (replacing nitrogen by oxygen) to thymidine, CpG sites are frequently mutated and become rare in the genome, except at CpG islands where they remain unmethylated. Epigenetic changes of this type thus have the potential to direct increased frequencies of permanent genetic mutation. DNA methylation patterns are known to be established and modified in response to environmental factors by a complex interplay of at least three independent DNA methyltransferases, DNMT1, DNMT3A, and DNMT3B, the loss of any of which is lethal in mice.[39] In invertebrate of social honey bees, main enzymes are DNMT1 and DNMT3.[40] DNMT1 is the most abundant methyltransferase in somatic cells,[41] localizes to replication foci,[42] has a 10–40-fold preference for hemimethylated DNA and interacts with the proliferating cell nuclear antigen (PCNA).[43]
By preferentially modifying hemimethylated DNA, DNMT1 transfers patterns of methylation to a newly synthesized strand after DNA replication, and therefore is often referred to as the 'maintenance' methyltransferase.[44] DNMT1 is essential for proper embryonic development, imprinting and X-inactivation.[39][45] To emphasize the difference of this molecular mechanism of inheritance from the canonical Watson-Crick base-pairing mechanism of transmission of genetic information, the term 'Epigenetic templating' was introduced.[46] Furthermore, in addition to the maintenance and transmission of methylated DNA states, the same principle could work in the maintenance and transmission of histone modifications and even cytoplasmic (structural) heritable states.[47]
In invertebrates of honey bees, DNA methylation has been studied since the honey bee genome [48] was sequenced in 2006. DNA methylation is associated with alternative splicing and gene regulation based on functional genomic research published in 2013.[49] In addition, DNA methylation is associated with the changes of expression in immune genes when honey bees were under lethal viral infection in a timely manner.[50] Several review papers have been published on the topics of DNA methylation in social insects.[51][52]
RNA methylation
RNA methylation of N6-methyladenosine (m6A) as the most abundant eukaryotic RNA modification has recently been recognized as an important gene regulatory mechanism.[53]
In invertebrates such as social insects of honey bees, RNA methylation is studied to be a possible epigenetic mechanism underlying aggression via reciprocal crosses.[54]
Histone modifications
Histones H3 and H4 can also be manipulated through demethylation using histone lysine demethylase (KDM). This recently identified enzyme has a catalytically active site called the Jumonji domain (JmjC). The demethylation occurs when JmjC utilizes multiple cofactors to hydroxylate the methyl group, thereby removing it. JmjC is capable of demethylating mono-, di-, and tri-methylated substrates.[55]
Chromosomal regions can adopt stable and heritable alternative states resulting in bistable gene expression without changes to the DNA sequence. Epigenetic control is often associated with alternative covalent modifications of histones.[56] The stability and heritability of states of larger chromosomal regions are suggested to involve positive feedback where modified nucleosomes recruit enzymes that similarly modify nearby nucleosomes.[57] A simplified stochastic model for this type of epigenetics is found here.[58][59]
It has been suggested that chromatin-based transcriptional regulation could be mediated by the effect of small RNAs. Small interfering RNAs can modulate transcriptional gene expression via epigenetic modulation of targeted promoters.[60]
RNA transcripts
Sometimes a gene, after being turned on, transcribes a product that (directly or indirectly) maintains the activity of that gene. For example, Hnf4 and MyoD enhance the transcription of many liver-specific and muscle-specific genes, respectively, including their own, through the transcription factor activity of the proteins they encode. RNA signalling includes differential recruitment of a hierarchy of generic chromatin modifying complexes and DNA methyltransferases to specific loci by RNAs during differentiation and development.[61] Other epigenetic changes are mediated by the production of different splice forms of RNA, or by formation of double-stranded RNA (RNAi). Descendants of the cell in which the gene was turned on will inherit this activity, even if the original stimulus for gene-activation is no longer present. These genes are often turned on or off by signal transduction, although in some systems where syncytia or gap junctions are important, RNA may spread directly to other cells or nuclei by diffusion. A large amount of RNA and protein is contributed to the zygote by the mother during oogenesis or via nurse cells, resulting in maternal effect phenotypes. A smaller quantity of sperm RNA is transmitted from the father, but there is recent evidence that this epigenetic information can lead to visible changes in several generations of offspring.[62]
MicroRNAs
MicroRNAs (miRNAs) are members of non-coding RNAs that range in size from 17 to 25 nucleotides. miRNAs regulate a large variety of biological functions in plants and animals.[63] So far, in 2013, about 2000 miRNAs have been discovered in humans and these can be found online in a miRNA database.[64] Each miRNA expressed in a cell may target about 100 to 200 messenger RNAs(mRNAs) that it downregulates.[65] Most of the downregulation of mRNAs occurs by causing the decay of the targeted mRNA, while some downregulation occurs at the level of translation into protein.[66]
It appears that about 60% of human protein coding genes are regulated by miRNAs.[67] Many miRNAs are epigenetically regulated. About 50% of miRNA genes are associated with CpG islands,[63] that may be repressed by epigenetic methylation. Transcription from methylated CpG islands is strongly and heritably repressed.[68] Other miRNAs are epigenetically regulated by either histone modifications or by combined DNA methylation and histone modification.[63]
mRNA
In 2011, it was demonstrated that the methylation of mRNA plays a critical role in human energy homeostasis. The obesity-associated FTO gene is shown to be able to demethylate N6-methyladenosine in RNA.[69][70]
sRNAs
sRNAs are small (50–250 nucleotides), highly structured, non-coding RNA fragments found in bacteria. They control gene expression including virulence genes in pathogens and are viewed as new targets in the fight against drug-resistant bacteria.[71] They play an important role in many biological processes, binding to mRNA and protein targets in prokaryotes. Their phylogenetic analyses, for example through sRNA–mRNA target interactions or protein binding properties, are used to build comprehensive databases.[72] sRNA-gene maps based on their targets in microbial genomes are also constructed.[73]
Long non-coding RNAs
Numerous investigations have demonstrated the pivotal involvement of long non-coding RNAs (lncRNAs) in the regulation of gene expression and chromosomal modifications, thereby exerting significant control over cellular differentiation. These long non-coding RNAs also contribute to genomic imprinting and the inactivation of the X chromosome.[74] In invertebrates such as social insects of honey bees, long non-coding RNAs are detected as a possible epigenetic mechanism via allele-specific genes underlying aggression via reciprocal crosses.[75]
Prions
Prions are infectious forms of proteins. In general, proteins fold into discrete units that perform distinct cellular functions, but some proteins are also capable of forming an infectious conformational state known as a prion. Although often viewed in the context of infectious disease, prions are more loosely defined by their ability to catalytically convert other native state versions of the same protein to an infectious conformational state. It is in this latter sense that they can be viewed as epigenetic agents capable of inducing a phenotypic change without a modification of the genome.[76]
Fungal prions are considered by some to be epigenetic because the infectious phenotype caused by the prion can be inherited without modification of the genome. PSI+ and URE3, discovered in yeast in 1965 and 1971, are the two best studied of this type of prion.[77][78] Prions can have a phenotypic effect through the sequestration of protein in aggregates, thereby reducing that protein's activity. In PSI+ cells, the loss of the Sup35 protein (which is involved in termination of translation) causes ribosomes to have a higher rate of read-through of stop codons, an effect that results in suppression of nonsense mutations in other genes.[79] The ability of Sup35 to form prions may be a conserved trait. It could confer an adaptive advantage by giving cells the ability to switch into a PSI+ state and express dormant genetic features normally terminated by stop codon mutations.[80][81][82][83]
Prion-based epigenetics has also been observed in Saccharomyces cerevisiae.[84]
Molecular basis
Epigenetic changes modify the activation of certain genes, but not the genetic code sequence of DNA.[85] The microstructure (not code) of DNA itself or the associated chromatin proteins may be modified, causing activation or silencing. This mechanism enables differentiated cells in a multicellular organism to express only the genes that are necessary for their own activity. Epigenetic changes are preserved when cells divide. Most epigenetic changes only occur within the course of one individual organism's lifetime; however, these epigenetic changes can be transmitted to the organism's offspring through a process called transgenerational epigenetic inheritance. Moreover, if gene inactivation occurs in a sperm or egg cell that results in fertilization, this epigenetic modification may also be transferred to the next generation.[86]
Specific epigenetic processes include paramutation, bookmarking, imprinting, gene silencing, X chromosome inactivation, position effect, DNA methylation reprogramming, transvection, maternal effects, the progress of carcinogenesis, many effects of teratogens, regulation of histone modifications and heterochromatin, and technical limitations affecting parthenogenesis and cloning.[87][88][89]
DNA damage
DNA damage can also cause epigenetic changes.[90][91][92] DNA damage is very frequent, occurring on average about 60,000 times a day per cell of the human body (see DNA damage (naturally occurring)). These damages are largely repaired, however, epigenetic changes can still remain at the site of DNA repair.[93] In particular, a double strand break in DNA can initiate unprogrammed epigenetic gene silencing both by causing DNA methylation as well as by promoting silencing types of histone modifications (chromatin remodeling - see next section).[94] In addition, the enzyme Parp1 (poly(ADP)-ribose polymerase) and its product poly(ADP)-ribose (PAR) accumulate at sites of DNA damage as part of the repair process.[95] This accumulation, in turn, directs recruitment and activation of the chromatin remodeling protein, ALC1, that can cause nucleosome remodeling.[96] Nucleosome remodeling has been found to cause, for instance, epigenetic silencing of DNA repair gene MLH1.[22][97] DNA damaging chemicals, such as benzene, hydroquinone, styrene, carbon tetrachloride and trichloroethylene, cause considerable hypomethylation of DNA, some through the activation of oxidative stress pathways.[98]
Foods are known to alter the epigenetics of rats on different diets.[99] Some food components epigenetically increase the levels of DNA repair enzymes such as MGMT and MLH1[100] and p53.[101][102] Other food components can reduce DNA damage, such as soy isoflavones. In one study, markers for oxidative stress, such as modified nucleotides that can result from DNA damage, were decreased by a 3-week diet supplemented with soy.[103] A decrease in oxidative DNA damage was also observed 2 h after consumption of anthocyanin-rich bilberry (Vaccinium myrtillius L.) pomace extract.[104]
DNA repair
Damage to DNA is very common and is constantly being repaired. Epigenetic alterations can accompany DNA repair of oxidative damage or double-strand breaks. In human cells, oxidative DNA damage occurs about 10,000 times a day and DNA double-strand breaks occur about 10 to 50 times a cell cycle in somatic replicating cells (see DNA damage (naturally occurring)). The selective advantage of DNA repair is to allow the cell to survive in the face of DNA damage. The selective advantage of epigenetic alterations that occur with DNA repair is not clear.[citation needed]
Repair of oxidative DNA damage can alter epigenetic markers
In the steady state (with endogenous damages occurring and being repaired), there are about 2,400 oxidatively damaged guanines that form 8-oxo-2'-deoxyguanosine (8-OHdG) in the average mammalian cell DNA.[105] 8-OHdG constitutes about 5% of the oxidative damages commonly present in DNA.[106] The oxidized guanines do not occur randomly among all guanines in DNA. There is a sequence preference for the guanine at a methylated CpG site (a cytosine followed by guanine along its 5' → 3' direction and where the cytosine is methylated (5-mCpG)).[107] A 5-mCpG site has the lowest ionization potential for guanine oxidation.Lua error: Internal error: The interpreter exited with status 1.
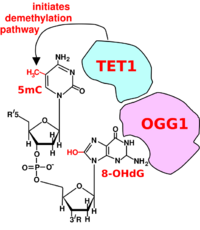
Oxidized guanine has mispairing potential and is mutagenic.[109] Oxoguanine glycosylase (OGG1) is the primary enzyme responsible for the excision of the oxidized guanine during DNA repair. OGG1 finds and binds to an 8-OHdG within a few seconds.[110] However, OGG1 does not immediately excise 8-OHdG. In HeLa cells half maximum removal of 8-OHdG occurs in 30 minutes,[111] and in irradiated mice, the 8-OHdGs induced in the mouse liver are removed with a half-life of 11 minutes.[106]
When OGG1 is present at an oxidized guanine within a methylated CpG site it recruits TET1 to the 8-OHdG lesion (see Figure). This allows TET1 to demethylate an adjacent methylated cytosine. Demethylation of cytosine is an epigenetic alteration.Lua error: Internal error: The interpreter exited with status 1.
As an example, when human mammary epithelial cells were treated with H2O2 for six hours, 8-OHdG increased about 3.5-fold in DNA and this caused about 80% demethylation of the 5-methylcytosines in the genome.[108] Demethylation of CpGs in a gene promoter by TET enzyme activity increases transcription of the gene into messenger RNA.[112] In cells treated with H2O2, one particular gene was examined, BACE1.[108] The methylation level of the BACE1 CpG island was reduced (an epigenetic alteration) and this allowed about 6.5 fold increase of expression of BACE1 messenger RNA.Lua error: Internal error: The interpreter exited with status 1.
While six-hour incubation with H2O2 causes considerable demethylation of 5-mCpG sites, shorter times of H2O2 incubation appear to promote other epigenetic alterations. Treatment of cells with H2O2 for 30 minutes causes the mismatch repair protein heterodimer MSH2-MSH6 to recruit DNA methyltransferase 1 (DNMT1) to sites of some kinds of oxidative DNA damage.[113] This could cause increased methylation of cytosines (epigenetic alterations) at these locations.
Jiang et al.[114] treated HEK 293 cells with agents causing oxidative DNA damage, (potassium bromate (KBrO3) or potassium chromate (K2CrO4)). Base excision repair (BER) of oxidative damage occurred with the DNA repair enzyme polymerase beta localizing to oxidized guanines. Polymerase beta is the main human polymerase in short-patch BER of oxidative DNA damage. Jiang et al.[114] also found that polymerase beta recruited the DNA methyltransferase protein DNMT3b to BER repair sites. They then evaluated the methylation pattern at the single nucleotide level in a small region of DNA including the promoter region and the early transcription region of the BRCA1 gene. Oxidative DNA damage from bromate modulated the DNA methylation pattern (caused epigenetic alterations) at CpG sites within the region of DNA studied. In untreated cells, CpGs located at −189, −134, −29, −19, +16, and +19 of the BRCA1 gene had methylated cytosines (where numbering is from the messenger RNA transcription start site, and negative numbers indicate nucleotides in the upstream promoter region). Bromate treatment-induced oxidation resulted in the loss of cytosine methylation at −189, −134, +16 and +19 while also leading to the formation of new methylation at the CpGs located at −80, −55, −21 and +8 after DNA repair was allowed.
Homologous recombinational repair alters epigenetic markers
At least four articles report the recruitment of DNA methyltransferase 1 (DNMT1) to sites of DNA double-strand breaks.[115][116][117][118] During homologous recombinational repair (HR) of the double-strand break, the involvement of DNMT1 causes the two repaired strands of DNA to have different levels of methylated cytosines. One strand becomes frequently methylated at about 21 CpG sites downstream of the repaired double-strand break. The other DNA strand loses methylation at about six CpG sites that were previously methylated downstream of the double-strand break, as well as losing methylation at about five CpG sites that were previously methylated upstream of the double-strand break. When the chromosome is replicated, this gives rise to one daughter chromosome that is heavily methylated downstream of the previous break site and one that is unmethylated in the region both upstream and downstream of the previous break site. With respect to the gene that was broken by the double-strand break, half of the progeny cells express that gene at a high level and in the other half of the progeny cells expression of that gene is repressed. When clones of these cells were maintained for three years, the new methylation patterns were maintained over that time period.[119]
In mice with a CRISPR-mediated homology-directed recombination insertion in their genome there were a large number of increased methylations of CpG sites within the double-strand break-associated insertion.[120]
Non-homologous end joining can cause some epigenetic marker alterations
Non-homologous end joining (NHEJ) repair of a double-strand break can cause a small number of demethylations of pre-existing cytosine DNA methylations downstream of the repaired double-strand break.[116] Further work by Allen et al.[121] showed that NHEJ of a DNA double-strand break in a cell could give rise to some progeny cells having repressed expression of the gene harboring the initial double-strand break and some progeny having high expression of that gene due to epigenetic alterations associated with NHEJ repair. The frequency of epigenetic alterations causing repression of a gene after an NHEJ repair of a DNA double-strand break in that gene may be about 0.9%.[117]
Techniques used to study epigenetics
Epigenetic research uses a wide range of molecular biological techniques to further understanding of epigenetic phenomena. These techniques include chromatin immunoprecipitation (together with its large-scale variants ChIP-on-chip and ChIP-Seq), fluorescent in situ hybridization, methylation-sensitive restriction enzymes, DNA adenine methyltransferase identification (DamID) and bisulfite sequencing.[122] Furthermore, the use of bioinformatics methods has a role in computational epigenetics.[122]
Chromatin Immunoprecipitation
Chromatin Immunoprecipitation (ChIP) has helped bridge the gap between DNA and epigenetic interactions.[123] With the use of ChIP, researchers are able to make findings in regards to gene regulation, transcription mechanisms, and chromatin structure.[123]
Fluorescent in situ hybridization
Fluorescent in situ hybridization (FISH) is very important to understand epigenetic mechanisms.[124] FISH can be used to find the location of genes on chromosomes, as well as finding noncoding RNAs.[124][125] FISH is predominantly used for detecting chromosomal abnormalities in humans.[125]
Methylation-sensitive restriction enzymes
Methylation sensitive restriction enzymes paired with PCR is a way to evaluate methylation in DNA - specifically the CpG sites.[126] If DNA is methylated, the restriction enzymes will not cleave the strand.[126] Contrarily, if the DNA is not methylated, the enzymes will cleave the strand and it will be amplified by PCR.[126]
Bisulfite sequencing
Bisulfite sequencing is another way to evaluate DNA methylation. Cytosine will be changed to uracil from being treated with sodium bisulfite, whereas methylated cytosines will not be affected.[126][50][49]
Nanopore sequencing
Certain sequencing methods, such as nanopore sequencing, allow sequencing of native DNA. Native (=unamplified) DNA retains the epigenetic modifications which would otherwise be lost during the amplification step. Nanopore basecaller models can distinguish between the signals obtained for epigenetically modified bases and unaltered based and provide an epigenetic profile in addition to the sequencing result.[127]
Structural inheritance
Lua error: Internal error: The interpreter exited with status 1. In ciliates such as Tetrahymena and Paramecium, genetically identical cells show heritable differences in the patterns of ciliary rows on their cell surface. Experimentally altered patterns can be transmitted to daughter cells. It seems existing structures act as templates for new structures. The mechanisms of such inheritance are unclear, but reasons exist to assume that multicellular organisms also use existing cell structures to assemble new ones.[128][129][130]
Nucleosome positioning
Eukaryotic genomes have numerous nucleosomes. Nucleosome position is not random, and determine the accessibility of DNA to regulatory proteins. Promoters active in different tissues have been shown to have different nucleosome positioning features.[131] This determines differences in gene expression and cell differentiation. It has been shown that at least some nucleosomes are retained in sperm cells (where most but not all histones are replaced by protamines). Thus nucleosome positioning is to some degree inheritable. Recent studies have uncovered connections between nucleosome positioning and other epigenetic factors, such as DNA methylation and hydroxymethylation.[132]
Histone variants
Different histone variants are incorporated into specific regions of the genome non-randomly. Their differential biochemical characteristics can affect genome functions via their roles in gene regulation,[133] and maintenance of chromosome structures.[134]
Genomic architecture
The three-dimensional configuration of the genome (the 3D genome) is complex, dynamic and crucial for regulating genomic function and nuclear processes such as DNA replication, transcription and DNA-damage repair.[135]
Functions and consequences
In the brain
Memory
Memory formation and maintenance are due to epigenetic alterations that cause the required dynamic changes in gene transcription that create and renew memory in neurons.[31]
An event can set off a chain of reactions that result in altered methylations of a large set of genes in neurons, which give a representation of the event, a memory.[31]
Areas of the brain important in the formation of memories include the hippocampus, medial prefrontal cortex (mPFC), anterior cingulate cortex and amygdala, as shown in the diagram of the human brain in this section.[136]
When a strong memory is created, as in a rat subjected to contextual fear conditioning (CFC), one of the earliest events to occur is that more than 100 DNA double-strand breaks are formed by topoisomerase IIB in neurons of the hippocampus and the medial prefrontal cortex (mPFC).[137] These double-strand breaks are at specific locations that allow activation of transcription of immediate early genes (IEGs) that are important in memory formation, allowing their expression in mRNA, with peak mRNA transcription at seven to ten minutes after CFC.[137][138]
Two important IEGs in memory formation are EGR1[139] and the alternative promoter variant of DNMT3A, DNMT3A2.[140] EGR1 protein binds to DNA at its binding motifs, 5′-GCGTGGGCG-3′ or 5′-GCGGGGGCGG-3', and there are about 12,000 genome locations at which EGR1 protein can bind.[141] EGR1 protein binds to DNA in gene promoter and enhancer regions. EGR1 recruits the demethylating enzyme TET1 to an association, and brings TET1 to about 600 locations on the genome where TET1 can then demethylate and activate the associated genes.[141]
The DNA methyltransferases DNMT3A1, DNMT3A2 and DNMT3B can all methylate cytosines (see image this section) at CpG sites in or near the promoters of genes. As shown by Manzo et al.,[142] these three DNA methyltransferases differ in their genomic binding locations and DNA methylation activity at different regulatory sites. Manzo et al. located 3,970 genome regions exclusively enriched for DNMT3A1, 3,838 regions for DNMT3A2 and 3,432 regions for DNMT3B. When DNMT3A2 is newly induced as an IEG (when neurons are activated), many new cytosine methylations occur, presumably in the target regions of DNMT3A2. Oliviera et al.[140] found that the neuronal activity-inducible IEG levels of Dnmt3a2 in the hippocampus determined the ability to form long-term memories.
Rats form long-term associative memories after contextual fear conditioning (CFC).[143] Duke et al.[30] found that 24 hours after CFC in rats, in hippocampus neurons, 2,097 genes (9.17% of the genes in the rat genome) had altered methylation. When newly methylated cytosines are present in CpG sites in the promoter regions of genes, the genes are often repressed, and when newly demethylated cytosines are present the genes may be activated.[144] After CFC, there were 1,048 genes with reduced mRNA expression and 564 genes with upregulated mRNA expression. Similarly, when mice undergo CFC, one hour later in the hippocampus region of the mouse brain there are 675 demethylated genes and 613 hypermethylated genes.[145] However, memories do not remain in the hippocampus, but after four or five weeks the memories are stored in the anterior cingulate cortex.[146] In the studies on mice after CFC, Halder et al.[145] showed that four weeks after CFC there were at least 1,000 differentially methylated genes and more than 1,000 differentially expressed genes in the anterior cingulate cortex, while at the same time the altered methylations in the hippocampus were reversed.
The epigenetic alteration of methylation after a new memory is established creates a different pool of nuclear mRNAs. As reviewed by Bernstein,[31] the epigenetically determined new mix of nuclear mRNAs are often packaged into neuronal granules, or messenger RNP, consisting of mRNA, small and large ribosomal subunits, translation initiation factors and RNA-binding proteins that regulate mRNA function. These neuronal granules are transported from the neuron nucleus and are directed, according to 3′ untranslated regions of the mRNA in the granules (their "zip codes"), to neuronal dendrites. Roughly 2,500 mRNAs may be localized to the dendrites of hippocampal pyramidal neurons and perhaps 450 transcripts are in excitatory presynaptic nerve terminals (dendritic spines). The altered assortments of transcripts (dependent on epigenetic alterations in the neuron nucleus) have different sensitivities in response to signals, which is the basis of altered synaptic plasticity. Altered synaptic plasticity is often considered the neurochemical foundation of learning and memory.
Aging
Epigenetics play a major role in brain aging and age-related cognitive decline, with relevance to life extension.[147][148][149][150][151]
Other and general
In adulthood, changes in the epigenome are important for various higher cognitive functions. Dysregulation of epigenetic mechanisms is implicated in neurodegenerative disorders and diseases. Epigenetic modifications in neurons are dynamic and reversible.[152] Epigenetic regulation impacts neuronal action, affecting learning, memory, and other cognitive processes.[153]
Early events, including during embryonic development, can influence development, cognition, and health outcomes through epigenetic mechanisms.[154]
Epigenetic mechanisms have been proposed as "a potential molecular mechanism for effects of endogenous hormones on the organization of developing brain circuits".[155]
Nutrients could interact with the epigenome to "protect or boost cognitive processes across the lifespan".[156][157]
A review suggests neurobiological effects of physical exercise via epigenetics seem "central to building an 'epigenetic memory' to influence long-term brain function and behavior" and may even be heritable.[158]
With the axo-ciliary synapse, there is communication between serotonergic axons and antenna-like primary cilia of CA1 pyramidal neurons that alters the neuron's epigenetic state in the nucleus via the signalling distinct from that at the plasma membrane (and longer-term).[159][160]
Epigenetics also play a major role in the brain evolution in and to humans.[161]
Development
Developmental epigenetics can be divided into predetermined and probabilistic epigenesis. Predetermined epigenesis is a unidirectional movement from structural development in DNA to the functional maturation of the protein. "Predetermined" here means that development is scripted and predictable. Probabilistic epigenesis on the other hand is a bidirectional structure-function development with experiences and external molding development.[162]
Somatic epigenetic inheritance, particularly through DNA and histone covalent modifications and nucleosome repositioning, is very important in the development of multicellular eukaryotic organisms.[132] The genome sequence is static (with some notable exceptions), but cells differentiate into many different types, which perform different functions, and respond differently to the environment and intercellular signaling. Thus, as individuals develop, morphogens activate or silence genes in an epigenetically heritable fashion, giving cells a memory. In mammals, most cells terminally differentiate, with only stem cells retaining the ability to differentiate into several cell types ("totipotency" and "multipotency"). In mammals, some stem cells continue producing newly differentiated cells throughout life, such as in neurogenesis, but mammals are not able to respond to loss of some tissues, for example, the inability to regenerate limbs, which some other animals are capable of. Epigenetic modifications regulate the transition from neural stem cells to glial progenitor cells (for example, differentiation into oligodendrocytes is regulated by the deacetylation and methylation of histones.[163] Unlike animals, plant cells do not terminally differentiate, remaining totipotent with the ability to give rise to a new individual plant. While plants do utilize many of the same epigenetic mechanisms as animals, such as chromatin remodeling, it has been hypothesized that some kinds of plant cells do not use or require "cellular memories", resetting their gene expression patterns using positional information from the environment and surrounding cells to determine their fate.[164]
Epigenetic changes can occur in response to environmental exposure – for example, maternal dietary supplementation with genistein (250 mg/kg) have epigenetic changes affecting expression of the agouti gene, which affects their fur color, weight, and propensity to develop cancer.[165][166][167] Ongoing research is focused on exploring the impact of other known teratogens, such as diabetic embryopathy, on methylation signatures.[168]
Controversial results from one study suggested that traumatic experiences might produce an epigenetic signal that is capable of being passed to future generations. Mice were trained, using foot shocks, to fear a cherry blossom odor. The investigators reported that the mouse offspring had an increased aversion to this specific odor.[169][170] They suggested epigenetic changes that increase gene expression, rather than in DNA itself, in a gene, M71, that governs the functioning of an odor receptor in the nose that responds specifically to this cherry blossom smell. There were physical changes that correlated with olfactory (smell) function in the brains of the trained mice and their descendants. Several criticisms were reported, including the study's low statistical power as evidence of some irregularity such as bias in reporting results.[171] Due to limits of sample size, there is a probability that an effect will not be demonstrated to within statistical significance even if it exists. The criticism suggested that the probability that all the experiments reported would show positive results if an identical protocol was followed, assuming the claimed effects exist, is merely 0.4%. The authors also did not indicate which mice were siblings, and treated all of the mice as statistically independent.[172] The original researchers pointed out negative results in the paper's appendix that the criticism omitted in its calculations, and undertook to track which mice were siblings in the future.[173]
Transgenerational
Epigenetic mechanisms were a necessary part of the evolutionary origin of cell differentiation.[174]Lua error: Internal error: The interpreter exited with status 1. Although epigenetics in multicellular organisms is generally thought to be a mechanism involved in differentiation, with epigenetic patterns "reset" when organisms reproduce, there have been some observations of transgenerational epigenetic inheritance (e.g., the phenomenon of paramutation observed in maize). Although most of these multigenerational epigenetic traits are gradually lost over several generations, the possibility remains that multigenerational epigenetics could be another aspect to evolution and adaptation. As mentioned above, some define epigenetics as heritable.
A sequestered germ line or Weismann barrier is specific to animals, and epigenetic inheritance is more common in plants and microbes. Eva Jablonka, Marion J. Lamb and Étienne Danchin have argued that these effects may require enhancements to the standard conceptual framework of the modern synthesis and have called for an extended evolutionary synthesis.[175][176][177] Other evolutionary biologists, such as John Maynard Smith, have incorporated epigenetic inheritance into population-genetics models[178] or are openly skeptical of the extended evolutionary synthesis (Michael Lynch).[179] Thomas Dickins and Qazi Rahman state that epigenetic mechanisms such as DNA methylation and histone modification are genetically inherited under the control of natural selection and therefore fit under the earlier "modern synthesis".[180]
Two important ways in which epigenetic inheritance can differ from traditional genetic inheritance, with important consequences for evolution, are:
- rates of epimutation can be much faster than rates of mutation[181]
- the epimutations are more easily reversible[182]
In plants, heritable DNA methylation mutations are 100,000 times more likely to occur compared to DNA mutations.[183] An epigenetically inherited element such as the PSI+ system can act as a "stop-gap", good enough for short-term adaptation that allows the lineage to survive for long enough for mutation and/or recombination to genetically assimilate the adaptive phenotypic change.[184] The existence of this possibility increases the evolvability of a species.
More than 100 cases of transgenerational epigenetic inheritance phenomena have been reported in a wide range of organisms, including prokaryotes, plants, and animals.[185] For instance, mourning-cloak butterflies will change color through hormone changes in response to experimentation of varying temperatures.[186]
The filamentous fungus Neurospora crassa is a prominent model system for understanding the control and function of cytosine methylation. In this organism, DNA methylation is associated with relics of a genome-defense system called RIP (repeat-induced point mutation) and silences gene expression by inhibiting transcription elongation.[187]
The yeast prion PSI is generated by a conformational change of a translation termination factor, which is then inherited by daughter cells. This can provide a survival advantage under adverse conditions, exemplifying epigenetic regulation which enables unicellular organisms to respond rapidly to environmental stress. Prions can be viewed as epigenetic agents capable of inducing a phenotypic change without modification of the genome.[188]
Direct detection of epigenetic marks in microorganisms is possible with single molecule real time sequencing, in which polymerase sensitivity allows for measuring methylation and other modifications as a DNA molecule is being sequenced.[189] Several projects have demonstrated the ability to collect genome-wide epigenetic data in bacteria.[190][191][192][193]
Epigenetics in bacteria
While epigenetics is of fundamental importance in eukaryotes, especially metazoans, it plays a different role in bacteria.[194] Most importantly, eukaryotes use epigenetic mechanisms primarily to regulate gene expression which bacteria rarely do. However, bacteria make widespread use of postreplicative DNA methylation for the epigenetic control of DNA-protein interactions. Bacteria also use DNA adenine methylation (rather than DNA cytosine methylation) as an epigenetic signal. DNA adenine methylation is important in bacteria virulence in organisms such as Escherichia coli, Salmonella, Vibrio, Yersinia, Haemophilus, and Brucella. In Alphaproteobacteria, methylation of adenine regulates the cell cycle and couples gene transcription to DNA replication. In Gammaproteobacteria, adenine methylation provides signals for DNA replication, chromosome segregation, mismatch repair, packaging of bacteriophage, transposase activity and regulation of gene expression.[188][195] There exists a genetic switch controlling Streptococcus pneumoniae (the pneumococcus) that allows the bacterium to randomly change its characteristics into six alternative states that could pave the way to improved vaccines. Each form is randomly generated by a phase variable methylation system. The ability of the pneumococcus to cause deadly infections is different in each of these six states. Similar systems exist in other bacterial genera.[196] In Bacillota such as Clostridioides difficile, adenine methylation regulates sporulation, biofilm formation and host-adaptation.[197]
Medicine
Epigenetics has many and varied potential medical applications.[198] In 2008, the National Institutes of Health announced that $190 million had been earmarked for epigenetics research over the next five years. In announcing the funding, government officials noted that epigenetics has the potential to explain mechanisms of aging, human development, and the origins of cancer, heart disease, mental illness, as well as several other conditions. Some investigators, like Randy Jirtle, Ph.D., of Duke University Medical Center, think epigenetics may ultimately turn out to have a greater role in disease than genetics.[199]
Twins
Direct comparisons of identical twins constitute an optimal model for interrogating environmental epigenetics. In the case of humans with different environmental exposures, monozygotic (identical) twins were epigenetically indistinguishable during their early years, while older twins had remarkable differences in the overall content and genomic distribution of 5-methylcytosine DNA and histone acetylation.[11] The twin pairs who had spent less of their lifetime together and/or had greater differences in their medical histories were those who showed the largest differences in their levels of 5-methylcytosine DNA and acetylation of histones H3 and H4.[200]
Dizygotic (fraternal) and monozygotic (identical) twins show evidence of epigenetic influence in humans.[200][201][202] DNA sequence differences that would be abundant in a singleton-based study do not interfere with the analysis. Environmental differences can produce long-term epigenetic effects, and different developmental monozygotic twin subtypes may be different with respect to their susceptibility to be discordant from an epigenetic point of view.[203]
A high-throughput study, which denotes technology that looks at extensive genetic markers, focused on epigenetic differences between monozygotic twins to compare global and locus-specific changes in DNA methylation and histone modifications in a sample of 40 monozygotic twin pairs.[200] In this case, only healthy twin pairs were studied, but a wide range of ages was represented, between 3 and 74 years. One of the major conclusions from this study was that there is an age-dependent accumulation of epigenetic differences between the two siblings of twin pairs. This accumulation suggests the existence of epigenetic "drift". Epigenetic drift is the term given to epigenetic modifications as they occur as a direct function with age. While age is a known risk factor for many diseases, age-related methylation has been found to occur differentially at specific sites along the genome. Over time, this can result in measurable differences between biological and chronological age. Epigenetic changes have been found to be reflective of lifestyle and may act as functional biomarkers of disease before clinical threshold is reached.[204]
A more recent study, where 114 monozygotic twins and 80 dizygotic twins were analyzed for the DNA methylation status of around 6000 unique genomic regions, concluded that epigenetic similarity at the time of blastocyst splitting may also contribute to phenotypic similarities in monozygotic co-twins. This supports the notion that microenvironment at early stages of embryonic development can be quite important for the establishment of epigenetic marks.[201] Congenital genetic disease is well understood and it is clear that epigenetics can play a role, for example, in the case of Angelman syndrome and Prader–Willi syndrome. These are normal genetic diseases caused by gene deletions or inactivation of the genes but are unusually common because individuals are essentially hemizygous because of genomic imprinting, and therefore a single gene knock out is sufficient to cause the disease, where most cases would require both copies to be knocked out.[205]
Genomic imprinting
Lua error: Internal error: The interpreter exited with status 1.
Some human disorders are associated with genomic imprinting, a phenomenon in mammals where the father and mother contribute different epigenetic patterns for specific genomic loci in their germ cells.[206] The best-known case of imprinting in human disorders is that of Angelman syndrome and Prader–Willi syndrome – both can be produced by the same genetic mutation, chromosome 15q partial deletion, and the particular syndrome that will develop depends on whether the mutation is inherited from the child's mother or from their father.[207] This is due to the presence of genomic imprinting in the region. Beckwith–Wiedemann syndrome is also associated with genomic imprinting, often caused by abnormalities in maternal genomic imprinting of a region on chromosome 11.Lua error: Internal error: The interpreter exited with status 1.
Methyl CpG-binding protein 2 (MeCP2) is a transcriptional regulator that must be phosphorylated before releasing from the BDNF promoter, allowing transcription. Rett syndrome is underlain by mutations in the MeCP2 gene despite no large-scale changes in expression of MeCP2 being found in microarray analyses. BDNF is downregulated in the MECP2 mutant resulting in Rett syndrome, as well as the increase of early neural senescence and accumulation of damaged DNA.[208]
In the Överkalix study, paternal (but not maternal) grandsons[209] of Swedish men who were exposed during preadolescence to famine in the 19th century were less likely to die of cardiovascular disease. If food was plentiful, then diabetes mortality in the grandchildren increased, suggesting that this was a transgenerational epigenetic inheritance.[210] The opposite effect was observed for females – the paternal (but not maternal) granddaughters of women who experienced famine while in the womb (and therefore while their eggs were being formed) lived shorter lives on average.[211]
Diabetic wound healing
Epigenetic modifications have given insight into the understanding of the pathophysiology of different disease conditions. Though, they are strongly associated with cancer, their role in other pathological conditions are of equal importance. It appears that the hyperglycaemic environment could imprint such changes at the genomic level, that macrophages are primed towards a pro-inflammatory state and could fail to exhibit any phenotypic alteration towards the pro-healing type. This phenomenon of altered Macrophage Polarization is mostly associated with all the diabetic complications in a clinical set-up. As of 2018, several reports reveal the relevance of different epigenetic modifications with respect to diabetic complications. Sooner or later, with the advancements in biomedical tools, the detection of such biomarkers as prognostic and diagnostic tools in patients could possibly emerge out as alternative approaches. It is noteworthy to mention here that the use of epigenetic modifications as therapeutic targets warrant extensive preclinical as well as clinical evaluation prior to use.[212]
Examples of drugs altering gene expression from epigenetic events
The use of beta-lactam antibiotics can alter glutamate receptor activity and the action of cyclosporine on multiple transcription factors. Additionally, lithium can impact autophagy of aberrant proteins, and opioid drugs via chronic use can increase the expression of genes associated with addictive phenotypes.[213]
In a groundbreaking 2003 report, Caspi and colleagues demonstrated that in a robust cohort of over one-thousand subjects assessed multiple times from preschool to adulthood, subjects who carried one or two copies of the short allele of the serotonin transporter promoter polymorphism exhibited higher rates of adult depression and suicidality when exposed to childhood maltreatment when compared to long allele homozygotes with equal ELS exposure.[214]
Parental nutrition, in utero exposure to stress or endocrine disrupting chemicals,[215] male-induced maternal effects such as the attraction of differential mate quality, and maternal as well as paternal age, and offspring gender could all possibly influence whether a germline epimutation is ultimately expressed in offspring and the degree to which intergenerational inheritance remains stable throughout posterity.[216] However, whether and to what extent epigenetic effects can be transmitted across generations remains unclear, particularly in humans.[217][218]
Addiction
Addiction is a disorder of the brain's reward system which arises through transcriptional and neuroepigenetic mechanisms and occurs over time from chronically high levels of exposure to an addictive stimulus (e.g., morphine, cocaine, sexual intercourse, gambling, etc.).[219][220][221][222] Transgenerational epigenetic inheritance of addictive phenotypes has been noted to occur in preclinical studies.[223][224] However, robust evidence in support of the persistence of epigenetic effects across multiple generations has yet to be established in humans; for example, an epigenetic effect of prenatal exposure to smoking that is observed in great-grandchildren who had not been exposed.[217]
Depression
Epigenetic inheritance of depression-related phenotypes has also been reported in a preclinical study.[225] Inheritance of paternal stress-induced traits across generations involved small non-coding RNA signals transmitted via the paternal germline.Lua error: Internal error: The interpreter exited with status 1.
SARS-CoV-2
Recent work had demonstrated that SARS-CoV-2 markedly disrupts host cell epigenetic regulation. Viral proteins dampen antiviral responses by mimicking critical regions of human histone proteins, according to the study. The paper showed that SARS-CoV-2 protein encoded by ORF8 (ORF8) functions as a histone mimic of the ARKS motifs in histone H3 to disrupt host cell epigenetic regulation. Histone mimicry allows viruses to disrupt the host cell's ability to regulate gene expression, and respond to infection effectively. The discovery might have implications for the treatment of COVID-19 since SARS-CoV-2, lacking ORF8, is associated with decreased severity of COVID-19.[226]
Research
The two forms of heritable information, namely genetic and epigenetic, are collectively called dual inheritance. Members of the APOBEC/AID family of cytosine deaminases may concurrently influence genetic and epigenetic inheritance using similar molecular mechanisms, and may be a point of crosstalk between these conceptually compartmentalized processes.[227]
Fluoroquinolone antibiotics induce epigenetic changes in mammalian cells through iron chelation. This leads to epigenetic effects through inhibition of α-ketoglutarate-dependent dioxygenases that require iron as a co-factor.[228]
Various pharmacological agents are applied for the production of induced pluripotent stem cells (iPSC) or maintain the embryonic stem cell (ESC) phenotypic via epigenetic approach. Adult stem cells like bone marrow stem cells have also shown a potential to differentiate into cardiac competent cells when treated with G9a histone methyltransferase inhibitor BIX01294.[229][230]
Cell plasticity, which is the adaptation of cells to stimuli without changes in their genetic code, requires epigenetic changes. These have been observed in cell plasticity in cancer cells during epithelial-to-mesenchymal transition[231] and also in immune cells, such as macrophages.[232] Interestingly, metabolic changes underly these adaptations, since various metabolites play crucial roles in the chemistry of epigenetic marks. This includes for instance alpha-ketoglutarate, which is required for histone demethylation, and acetyl-Coenzyme A, which is required for histone acetylation.
Epigenome editing
Epigenetic regulation of gene expression that could be altered or used in epigenome editing are or include mRNA/lncRNA modification, DNA methylation modification and histone modification.[233][234][235]
CpG sites, SNPs and biological traits
Methylation is a widely characterized mechanism of genetic regulation that can determine biological traits. However, strong experimental evidences correlate methylation patterns in SNPs as an important additional feature for the classical activation/inhibition epigenetic dogma. Molecular interaction data, supported by colocalization analyses, identify multiple nuclear regulatory pathways, linking sequence variation to disturbances in DNA methylation and molecular and phenotypic variation.[236]
UBASH3B locus
UBASH3B encodes a protein with tyrosine phosphatase activity, which has been previously linked to advanced neoplasia.[237] SNP rs7115089 was identified as influencing DNA methylation and expression of this locus, as well as and Body Mass Index (BMI).[236] In fact, SNP rs7115089 is strongly associated with BMI[238] and with genetic variants linked to other cardiovascular and metabolic traits in GWASs.[239][240][241] New studies suggesting UBASH3B as a potential mediator of adiposity and cardiometabolic disease.[236] In addition, animal models demonstrated that UBASH3B expression is an indicator of caloric restriction that may drive programmed susceptibility to obesity and it is associated with other measures of adiposity in human peripherical blood.[242]
NFKBIE locus
SNP rs730775 is located in the first intron of NFKBIE and is a cis eQTL for NFKBIE in whole blood.[236] Nuclear factor (NF)-κB inhibitor ε (NFKBIE) directly inhibits NF-κB1 activity and is significantly co-expressed with NF-κB1, also, it is associated with rheumatoid arthritis.[243] Colocalization analysis supports that variants for the majority of the CpG sites in SNP rs730775 cause genetic variation at the NFKBIE locus which is suggestible linked to rheumatoid arthritis through trans acting regulation of DNA methylation by NF-κB.[236]
FADS1 locus
Fatty acid desaturase 1 (FADS1) is a key enzyme in the metabolism of fatty acids.[244] Moreover, rs174548 in the FADS1 gene shows increased correlation with DNA methylation in people with high abundance of CD8+ T cells.[236] SNP rs174548 is strongly associated with concentrations of arachidonic acid and other metabolites in fatty acid metabolism,[245][246] blood eosinophil counts.[247] and inflammatory diseases such as asthma.[248] Interaction results indicated a correlation between rs174548 and asthma, providing new insights about fatty acid metabolism in CD8+ T cells with immune phenotypes.[236]
Pseudoscience
As epigenetics is in the early stages of development as a science and is surrounded by sensationalism in the public media, David Gorski and geneticist Adam Rutherford have advised caution against the proliferation of false and pseudoscientific conclusions by new age authors making unfounded suggestions that a person's genes and health can be manipulated by mind control. Misuse of the scientific term by quack authors has produced misinformation among the general public.[2][249]
See also
- Baldwin effect
- Behavioral epigenetics
- Biological effects of radiation on the epigenome
- Computational epigenetics
- Contribution of epigenetic modifications to evolution
- DAnCER database (2010)
- Epigenesis
- Epigenetics in forensic science
- Epigenetic therapy
- Epigenetics of neurodegenerative diseases
- Genetics
- Lamarckism
- Nutriepigenomics
- Position-effect variegation
- Preformationism
- Somatic epitype
- Synthetic genetic array
- Sleep epigenetics
- Transcriptional memory
- Transgenerational epigenetic inheritance
References
- ↑ "Epigenetics: definition, mechanisms and clinical perspective". Seminars in Reproductive Medicine 27 (5): 351–7. September 2009. doi:10.1055/s-0029-1237423. PMID 19711245. "In the original sense of this definition, epigenetics referred to all molecular pathways modulating the expression of a genotype into a particular phenotype. Over the following years, with the rapid growth of genetics, the meaning of the word has gradually narrowed. Epigenetics has been defined and today is generally accepted as 'the study of changes in gene function that are mitotically and/or meiotically heritable and that do not entail a change in DNA sequence.'".
- ↑ 2.0 2.1 "Beware the pseudo gene genies". The Guardian. 19 July 2015. https://www.theguardian.com/science/2015/jul/19/epigenetics-dna--darwin-adam-rutherford.
- ↑ "What do you mean, "epigenetic"?". Genetics 199 (4): 887–896. April 2015. doi:10.1534/genetics.114.173492. PMID 25855649.
- ↑ "Epigenetics in cancer". Carcinogenesis 31 (1): 27–36. January 2010. doi:10.1093/carcin/bgp220. PMID 19752007.
- ↑ "Epigenetic modifications in cancer". Clinical Genetics 81 (4): 303–311. April 2012. doi:10.1111/j.1399-0004.2011.01809.x. PMID 22082348.
- ↑ "The Importance of ncRNAs as Epigenetic Mechanisms in Phenotypic Variation and Organic Evolution". Frontiers in Microbiology 8: 2483. 2017. doi:10.3389/fmicb.2017.02483. PMID 29312192.
- ↑ 7.0 7.1 "Perceptions of epigenetics". Nature 447 (7143): 396–398. May 2007. doi:10.1038/nature05913. PMID 17522671. Bibcode: 2007Natur.447..396B.
- ↑ "What genes remember". 1 May 2008. https://www.prospectmagazine.co.uk/magazine/whatgenesremember.
- ↑ "Stability and flexibility of epigenetic gene regulation in mammalian development". Nature 447 (7143): 425–32. May 2007. doi:10.1038/nature05918. PMID 17522676. Bibcode: 2007Natur.447..425R.
- ↑ Oxford English Dictionary: "The word is used by W. Harvey, Exercitationes 1651, p. 148, and in the English Anatomical Exercitations 1653, p. 272. It is explained to mean ‘partium super-exorientium additamentum’, ‘the additament of parts budding one out of another’."
- ↑ 11.0 11.1 The Developing Genome: An Introduction to Behavioral Epigenetics (1st ed.). Oxford University Press. 2015. ISBN 978-0199922345. https://global.oup.com/academic/product/the-developing-genome-9780199922345?cc=us&lang=en&.
- ↑ 12.0 12.1 "An operational definition of epigenetics". Genes & Development 23 (7): 781–3. April 2009. doi:10.1101/gad.1787609. PMID 19339683.
- ↑ 13.0 13.1 "Overview". NIH Roadmap Epigenomics Project. http://www.roadmapepigenomics.org/overview.
- ↑ Morange M. La tentative de Nikolai Koltzoff (Koltsov) de lier génétique, embryologie et chimie physique, J. Biosciences. 2011. V. 36. P. 211-214
- ↑ "The epigenotype". Endeavour 1: 18–20. 1942. "For the purpose of a study of inheritance, the relation between phenotypes and genotypes [...] is, from a wider biological point of view, of crucial importance, since it is the kernel of the whole problem of development. Many geneticists have recognized this and attempted to discover the processes involved in the mechanism by which the genes of the genotype bring about phenotypic effects. The first step in such an enterprise is – or rather should be, since it is often omitted by those with undue respect for the powers of reason – to describe what can be seen of the developmental processes. For enquiries of this kind, the word 'phenogenetics' was coined by Haecker [1918, Lua error: Internal error: The interpreter exited with status 1.]. The second and more important part of the task is to discover the causal mechanisms at work and to relate them as far as possible to what experimental embryology has already revealed of the mechanics of development. We might use the name 'epigenetics' for such studies, thus emphasizing their relation to the concepts, so strongly favourable to the classical theory of epigenesis, which have been reached by the experimental embryologists. We certainly need to remember that between genotype and phenotype, and connecting them to each other, there lies a whole complex of developmental processes. It is convenient to have a name for this complex: 'epigenotype' seems suitable."
- ↑ See preformationism for historical background. Oxford English Dictionary: "the theory that the germ is brought into existence (by successive accretions), and not merely developed, in the process of reproduction. [...] The opposite theory was formerly known as the 'theory of evolution'; to avoid the ambiguity of this name, it is now spoken of chiefly as the 'theory of preformation', sometimes as that of 'encasement' or 'emboîtement'."
- ↑ The Epigenetics of Birds. Cambridge University Press. 2014. ISBN 978-1-107-44047-0. https://books.google.com/books?id=lqKTBQAAQBAJ.Lua error: Internal error: The interpreter exited with status 1.
- ↑ "In search of evolutionary developmental mechanisms: the 30-year gap between 1944 and 1974". Journal of Experimental Zoology Part B: Molecular and Developmental Evolution 302 (1): 5–18. January 2004. doi:10.1002/jez.b.20002. PMID 14760651. Bibcode: 2004JEZ...302....5H.
- ↑ "Floral morphogenesis: stochastic explorations of a gene network epigenetic landscape". PLOS ONE 3 (11): e3626. 3 November 2008. doi:10.1371/journal.pone.0003626. PMID 18978941. Bibcode: 2008PLoSO...3.3626A.
- ↑ 20.0 20.1 "Branching and oscillations in the epigenetic landscape of cell-fate determination". Progress in Biophysics and Molecular Biology 117 (2–3): 240–249. March 2015. doi:10.1016/j.pbiomolbio.2015.01.006. PMID 25641423.
- ↑ "DNA methylation and epigenetic inheritance". Philosophical Transactions of the Royal Society of London. Series B, Biological Sciences 326 (1235): 329–38. January 1990. doi:10.1098/rstb.1990.0015. PMID 1968668. Bibcode: 1990RSPTB.326..329H.
- ↑ 22.0 22.1 Epigenetic mechanisms of gene regulation. Plainview, NY: Cold Spring Harbor Laboratory Press. 1996. pp. 1–4. ISBN 978-0-87969-490-6.Lua error: Internal error: The interpreter exited with status 1.
- ↑ "Language: Disputed definitions". Nature 455 (7216): 1023–8. October 2008. doi:10.1038/4551023a. PMID 18948925.
- ↑ "Epigenetics and gene expression". Heredity 105 (1): 4–13. July 2010. doi:10.1038/hdy.2010.54. PMID 20461105.
- ↑ "On the use of the word 'epigenetic'". Current Biology 17 (7): R233-6. April 2007. doi:10.1016/j.cub.2007.02.030. PMID 17407749.
- ↑ "Metabolic regulation of gene expression by histone lactylation". Nature 574 (7779): 575–580. October 2019. doi:10.1038/s41586-019-1678-1. PMID 31645732. Bibcode: 2019Natur.574..575Z.
- ↑ "Epigenetics of Modified DNA Bases: 5-Methylcytosine and Beyond". Frontiers in Genetics 9: 640. 2018. doi:10.3389/fgene.2018.00640. PMID 30619465.
- ↑ "The diverse roles of DNA methylation in mammalian development and disease". Nature Reviews. Molecular Cell Biology 20 (10): 590–607. October 2019. doi:10.1038/s41580-019-0159-6. PMID 31399642.
- ↑ "Transcription factors: from enhancer binding to developmental control". Nat Rev Genet 13 (9): 613–26. September 2012. doi:10.1038/nrg3207. PMID 22868264.
- ↑ 30.0 30.1 "Experience-dependent epigenomic reorganization in the hippocampus". Learn Mem 24 (7): 278–288. July 2017. doi:10.1101/lm.045112.117. PMID 28620075.
- ↑ 31.0 31.1 31.2 31.3 "DNA Methylation and Establishing Memory". Epigenet Insights 15: 25168657211072499. 2022. doi:10.1177/25168657211072499. PMID 35098021.
- ↑ 32.0 32.1 "Understanding the relationship between DNA methylation and histone lysine methylation". Biochim Biophys Acta 1839 (12): 1362–72. December 2014. doi:10.1016/j.bbagrm.2014.02.007. PMID 24560929.
- ↑ 33.0 33.1 "The interplay between DNA and histone methylation: molecular mechanisms and disease implications". EMBO Rep 22 (5): e51803. May 2021. doi:10.15252/embr.202051803. PMID 33844406.
- ↑ "The role of histone tails in nucleosome stability: An electrostatic perspective". Comput Struct Biotechnol J 18: 2799–2809. 2020. doi:10.1016/j.csbj.2020.09.034. PMID 33133421.
- ↑ "Relationship between histone H3 lysine 9 methylation, transcription repression, and heterochromatin protein 1 recruitment". Molecular and Cellular Biology 25 (7): 2525–2538. April 2005. doi:10.1128/MCB.25.7.2525-2538.2005. PMID 15767660.
- ↑ Biotechnology in Medical Sciences. United States: CRC Press (imprint of Taylor & Francis Group, an Informa business). 2014. pp. 239. ISBN 978-1-4822-2368-2.
- ↑ "SET domain proteins modulate chromatin domains in eu- and heterochromatin". Cellular and Molecular Life Sciences 54 (1): 80–93. January 1998. doi:10.1007/s000180050127. PMID 9487389.
- ↑ "Transposable elements and the epigenetic regulation of the genome". Nature Reviews. Genetics 8 (4): 272–85. April 2007. doi:10.1038/nrg2072. PMID 17363976.
- ↑ 39.0 39.1 "Targeted mutation of the DNA methyltransferase gene results in embryonic lethality". Cell 69 (6): 915–26. June 1992. doi:10.1016/0092-8674(92)90611-F. PMID 1606615.
- ↑ Li-Byarlay, "The function of DNA methylation marks in social insects", Frontiers in Ecology and Evolution 4, 57 https://www.frontiersin.org/articles/10.3389/fevo.2016.00057/full
- ↑ "The human DNA methyltransferases (DNMTs) 1, 3a and 3b: coordinate mRNA expression in normal tissues and overexpression in tumors". Nucleic Acids Research 27 (11): 2291–8. June 1999. doi:10.1093/nar/27.11.2291. PMID 10325416.
- ↑ "A targeting sequence directs DNA methyltransferase to sites of DNA replication in mammalian nuclei". Cell 71 (5): 865–73. November 1992. doi:10.1016/0092-8674(92)90561-P. PMID 1423634. https://epub.ub.uni-muenchen.de/5003/1/003.pdf.
- ↑ "Human DNA-(cytosine-5) methyltransferase-PCNA complex as a target for p21WAF1". Science 277 (5334): 1996–2000. September 1997. doi:10.1126/science.277.5334.1996. PMID 9302295.
- ↑ "DNA methylation in health and disease". Nature Reviews. Genetics 1 (1): 11–9. October 2000. doi:10.1038/35049533. PMID 11262868.
- ↑ "Role for DNA methylation in genomic imprinting". Nature 366 (6453): 362–5. November 1993. doi:10.1038/366362a0. PMID 8247133. Bibcode: 1993Natur.366..362L.
- ↑ "Analysis of human histone H2AZ deposition in vivo argues against its direct role in epigenetic templating mechanisms". Molecular and Cellular Biology 26 (14): 5325–35. July 2006. doi:10.1128/MCB.00584-06. PMID 16809769.
- ↑ "Erwin Schroedinger, Francis Crick and epigenetic stability". Biology Direct 3: 15. April 2008. doi:10.1186/1745-6150-3-15. PMID 18419815.
- ↑ The Honeybee Genome Sequencing Consortium The Honeybee Genome Sequencing Consortium
- ↑ 49.0 49.1 Li-Byarlay et al., "RNA interference knockdown of DNA methyl-transferase 3 affects gene alternative splicing in the honey bee", Proceedings of the National Academy of Sciences 110 (31), 12750-12755, https://doi.org/10.1073/pnas.1310735110
- ↑ 50.0 50.1 Li-Byarlay et al. 2020, "Transcriptomic and epigenomic dynamics of honey bees in response to lethal viral infection" Frontiers in genetics 11, 1056 https://doi.org/10.3389/fgene.2020.566320
- ↑ Li-Byarlay, "The Function of DNA Methylation Marks in Social Insects" Front. Ecol. Evol., 19 May 2016 Sec. Social Evolution, Volume 4 - 2016 https://doi.org/10.3389/fevo.2016.00057
- ↑ Wang & Li-Byarlay, "Chapter Two-Physiological and Molecular Mechanisms of Nutrition in Honey Bees", 2015, Advances in Insect Physiology, 49: 25-58. https://www.sciencedirect.com/science/article/abs/pii/S0065280615000259
- ↑ Barbieri I, Kouzarides T. Role of RNA modifications in cancer. Nat Rev Cancer. 2020;20(6):303–22.
- ↑ Bresnahan et al, "Examining parent-of-origin effects on transcription and RNA methylation in mediating aggressive behavior in honey bees (Apis mellifera)" BMC Genomics 24: 315 (2023), https://doi.org/10.1186/s12864-023-09411-4
- ↑ "Developmental roles of the histone lysine demethylases". Development 136 (6): 879–89. March 2009. doi:10.1242/dev.020966. PMID 19234061.
- ↑ "Determination of enriched histone modifications in non-genic portions of the human genome". BMC Genomics 10: 143. March 2009. doi:10.1186/1471-2164-10-143. PMID 19335899.
- ↑ "Ultrasensitive gene regulation by positive feedback loops in nucleosome modification". Molecular Systems Biology 4 (1): 182. 15 April 2008. doi:10.1038/msb.2008.21. PMID 18414483.
- ↑ "Epigenetic cell memory". Cmol.nbi.dk. http://cmol.nbi.dk/models/epigen/Epigen.html.
- ↑ "Theoretical analysis of epigenetic cell memory by nucleosome modification". Cell 129 (4): 813–22. May 2007. doi:10.1016/j.cell.2007.02.053. PMID 17512413.
- ↑ "Epigenetic Regulation of Gene Expression". RNA and the Regulation of Gene Expression: A Hidden Layer of Complexity. Norfolk, England: Caister Academic Press. 2008. ISBN 978-1-904455-25-7.Lua error: Internal error: The interpreter exited with status 1.
- ↑ "RNA regulation of epigenetic processes". BioEssays 31 (1): 51–9. January 2009. doi:10.1002/bies.080099. PMID 19154003.
- ↑ "RNA can be hereditary molecule". 25 May 2006. http://www.the-scientist.com/news/display/23494/.
- ↑ 63.0 63.1 63.2 "Transcriptional and epigenetic regulation of human microRNAs". Cancer Letters 331 (1): 1–10. April 2013. doi:10.1016/j.canlet.2012.12.006. PMID 23246373.
- ↑ "Browse miRBase by species". http://www.mirbase.org/cgi-bin/browse.pl.
- ↑ "Microarray analysis shows that some microRNAs downregulate large numbers of target mRNAs". Nature 433 (7027): 769–73. February 2005. doi:10.1038/nature03315. PMID 15685193. Bibcode: 2005Natur.433..769L.
- ↑ "MicroRNA-target interactions: new insights from genome-wide approaches". Annals of the New York Academy of Sciences 1271 (1): 118–28. October 2012. doi:10.1111/j.1749-6632.2012.06745.x. PMID 23050973. Bibcode: 2012NYASA1271..118L.
- ↑ "Most mammalian mRNAs are conserved targets of microRNAs". Genome Research 19 (1): 92–105. January 2009. doi:10.1101/gr.082701.108. PMID 18955434.
- ↑ "Eukaryotic cytosine methyltransferases". Annual Review of Biochemistry 74: 481–514. 2005. doi:10.1146/annurev.biochem.74.010904.153721. PMID 15952895.
- ↑ "N6-methyladenosine in nuclear RNA is a major substrate of the obesity-associated FTO". Nature Chemical Biology 7 (12): 885–7. October 2011. doi:10.1038/nchembio.687. PMID 22002720.
- ↑ "New research links common RNA modification to obesity". Physorg.com. http://www.physorg.com/news/2011-10-links-common-rna-modification-obesity.html.
- ↑ "Analysis of the small RNA transcriptional response in multidrug-resistant Staphylococcus aureus after antimicrobial exposure". Antimicrobial Agents and Chemotherapy 57 (8): 3864–74. August 2013. doi:10.1128/AAC.00263-13. PMID 23733475.
- ↑ "sRNATarBase 2.0 A comprehensive database of bacterial SRNA targets verified by experiments". http://ccb.bmi.ac.cn/srnatarbase/.
- ↑ "Genomics maps for small non-coding RNA's and their targets in microbial genomes". http://srnamap.mbc.nctu.edu.tw/.
- ↑ Ruffo, Paola, et al. "Long-noncoding RNAs as epigenetic regulators in neurodegenerative diseases." Neural Regeneration Research 18.6 (2023): 1243.
- ↑ Bresnahan et al, "Examining parent-of-origin effects on transcription and RNA methylation in mediating aggressive behavior in honey bees (Apis mellifera)" BMC Genomics volume 24, Article number: 315 (2023), https://doi.org/10.1186/s12864-023-09411-4
- ↑ "Epigenetic inheritance and prions". Journal of Evolutionary Biology 11 (2): 241–42. 1998. doi:10.1007/s000360050085.
- ↑ "[PSI], a cytoplasmic suppressor of super-suppression in yeast". Heredity 20 (4): 505–21. 1965. doi:10.1038/hdy.1965.65.
- ↑ "Non-Mendelian mutation allowing ureidosuccinic acid uptake in yeast". Journal of Bacteriology 106 (2): 519–22. May 1971. doi:10.1128/JB.106.2.519-522.1971. PMID 5573734.
- ↑ "Extrachromosomal psi+ determinant suppresses nonsense mutations in yeast". Journal of Bacteriology 139 (3): 1068–71. September 1979. doi:10.1128/JB.139.3.1068-1071.1979. PMID 225301.
- ↑ "A yeast prion provides a mechanism for genetic variation and phenotypic diversity". Nature 407 (6803): 477–83. September 2000. doi:10.1038/35035005. PMID 11028992. Bibcode: 2000Natur.407..477T.
- ↑ "Prions as adaptive conduits of memory and inheritance". Nature Reviews. Genetics 6 (6): 435–50. June 2005. doi:10.1038/nrg1616. PMID 15931169.
- ↑ "The conversion of 3' UTRs into coding regions". Molecular Biology and Evolution 24 (2): 457–64. February 2007. doi:10.1093/molbev/msl172. PMID 17099057.
- ↑ "The spontaneous appearance rate of the yeast prion [PSI+ and its implications for the evolution of the evolvability properties of the [PSI+] system"]. Genetics 184 (2): 393–400. February 2010. doi:10.1534/genetics.109.110213. PMID 19917766.
- ↑ "A prion accelerates proliferation at the expense of lifespan". eLife 10: e60917. September 2021. doi:10.7554/eLife.60917. PMID 34545808.
- ↑ "Wandering along the epigenetic timeline". Clin Epigenetics 12 (1): 97. July 2020. doi:10.1186/s13148-020-00893-7. PMID 32616071.
- ↑ "Paramutation: from maize to mice". Cell 128 (4): 641–5. February 2007. doi:10.1016/j.cell.2007.02.007. PMID 17320501.
- ↑ "Mitotic Gene Bookmarking: An Epigenetic Mechanism for Coordination of Lineage Commitment, Cell Identity and Cell Growth". RUNX Proteins in Development and Cancer. Advances in Experimental Medicine and Biology. 962. 2017. pp. 95–102. doi:10.1007/978-981-10-3233-2_7. ISBN 978-981-10-3231-8.
- ↑ "Paramutation: the tip of an epigenetic iceberg?". Trends in Genetics 26 (1): 9–14. January 2010. doi:10.1016/j.tig.2009.11.003. PMID 19945764.
- ↑ "Genomic imprinting: the emergence of an epigenetic paradigm". Nature Reviews. Genetics 12 (8): 565–575. July 2011. doi:10.1038/nrg3032. PMID 21765458.
- ↑ "Epigenetic changes and nontargeted radiation effects--is there a link?". Environmental and Molecular Mutagenesis 49 (1): 16–25. January 2008. doi:10.1002/em.20361. PMID 18172877. Bibcode: 2008EnvMM..49...16K.
- ↑ "Non-targeted radiation effects-an epigenetic connection". Mutation Research 714 (1–2): 113–25. September 2011. doi:10.1016/j.mrfmmm.2011.06.014. PMID 21784089.
- ↑ "Radiation-induced alterations in histone modification patterns and their potential impact on short-term radiation effects". Frontiers in Oncology 2: 117. 2012. doi:10.3389/fonc.2012.00117. PMID 23050241.
- ↑ "DNA damage, homology-directed repair, and DNA methylation". PLOS Genetics 3 (7): e110. July 2007. doi:10.1371/journal.pgen.0030110. PMID 17616978.
- ↑ "Double strand breaks can initiate gene silencing and SIRT1-dependent onset of DNA methylation in an exogenous promoter CpG island". PLOS Genetics 4 (8): e1000155. August 2008. doi:10.1371/journal.pgen.1000155. PMID 18704159.
- ↑ "The role of poly(ADP-ribose) in the DNA damage signaling network". Biochemistry and Cell Biology 83 (3): 354–64. June 2005. doi:10.1139/o05-038. PMID 15959561. https://www.zora.uzh.ch/id/eprint/5838/1/RPViewDoc.pdf.
- ↑ "Poly(ADP-ribosyl)ation directs recruitment and activation of an ATP-dependent chromatin remodeler". Proceedings of the National Academy of Sciences of the United States of America 106 (33): 13770–4. August 2009. doi:10.1073/pnas.0906920106. PMID 19666485. Bibcode: 2009PNAS..10613770G.
- ↑ "Role of nucleosomal occupancy in the epigenetic silencing of the MLH1 CpG island". Cancer Cell 12 (5): 432–44. November 2007. doi:10.1016/j.ccr.2007.10.014. PMID 17996647.
- ↑ "Epigenetic factors in cancer risk: effect of chemical carcinogens on global DNA methylation pattern in human TK6 cells". PLOS ONE 7 (4): e34674. 2012. doi:10.1371/journal.pone.0034674. PMID 22509344. Bibcode: 2012PLoSO...734674T.
- ↑ "Progressive, transgenerational changes in offspring phenotype and epigenotype following nutritional transition". PLOS ONE 6 (11): e28282. 2011. doi:10.1371/journal.pone.0028282. PMID 22140567. Bibcode: 2011PLoSO...628282B.
- ↑ "Dietary polyphenols may affect DNA methylation". The Journal of Nutrition 137 (1 Suppl): 223S–228S. January 2007. doi:10.1093/jn/137.1.223S. PMID 17182830.
- ↑ "The flavoring agent dihydrocoumarin reverses epigenetic silencing and inhibits sirtuin deacetylases". PLOS Genetics 1 (6): e77. December 2005. doi:10.1371/journal.pgen.0010077. PMID 16362078.
- ↑ "Genistein mediated histone acetylation and demethylation activates tumor suppressor genes in prostate cancer cells". International Journal of Cancer 123 (3): 552–60. August 2008. doi:10.1002/ijc.23590. PMID 18431742.
- ↑ "Effect of soy isoflavone supplementation on markers of oxidative stress in men and women". Cancer Letters 172 (1): 1–6. October 2001. doi:10.1016/S0304-3835(01)00627-9. PMID 11595123.
- ↑ "Modulation of Nrf2-dependent gene transcription by bilberry anthocyanins in vivo". Molecular Nutrition & Food Research 57 (3): 545–50. March 2013. doi:10.1002/mnfr.201200504. PMID 23349102.
- ↑ "Endogenous versus exogenous DNA adducts: their role in carcinogenesis, epidemiology, and risk assessment". Toxicol Sci 120 (Suppl 1): S130–45. March 2011. doi:10.1093/toxsci/kfq371. PMID 21163908.
- ↑ 106.0 106.1 "A reliable assessment of 8-oxo-2-deoxyguanosine levels in nuclear and mitochondrial DNA using the sodium iodide method to isolate DNA". Nucleic Acids Res 29 (10): 2117–26. May 2001. doi:10.1093/nar/29.10.2117. PMID 11353081.
- ↑ "Mapping structurally defined guanine oxidation products along DNA duplexes: influence of local sequence context and endogenous cytosine methylation". J Am Chem Soc 136 (11): 4223–35. March 2014. doi:10.1021/ja411636j. PMID 24571128.
- ↑ 108.0 108.1 108.2 "OGG1 is essential in oxidative stress-induced DNA demethylation". Cell Signal 28 (9): 1163–1171. September 2016. doi:10.1016/j.cellsig.2016.05.021. PMID 27251462.
- ↑ "The genomics of oxidative DNA damage, repair, and resulting mutagenesis". Comput Struct Biotechnol J 18: 207–219. 2020. doi:10.1016/j.csbj.2019.12.013. PMID 31993111.
- ↑ "Lost in the Crowd: How Does Human 8-Oxoguanine DNA Glycosylase 1 (OGG1) Find 8-Oxoguanine in the Genome?". Int J Mol Sci 21 (21): 8360. November 2020. doi:10.3390/ijms21218360. PMID 33171795.
- ↑ "In situ analysis of repair processes for oxidative DNA damage in mammalian cells". Proc Natl Acad Sci U S A 101 (38): 13738–43. September 2004. doi:10.1073/pnas.0406048101. PMID 15365186. Bibcode: 2004PNAS..10113738L.
- ↑ "Targeted DNA demethylation and activation of endogenous genes using programmable TALE-TET1 fusion proteins". Nat. Biotechnol. 31 (12): 1137–42. December 2013. doi:10.1038/nbt.2726. PMID 24108092.
- ↑ "Mismatch repair proteins recruit DNA methyltransferase 1 to sites of oxidative DNA damage". J Mol Cell Biol 8 (3): 244–54. June 2016. doi:10.1093/jmcb/mjv050. PMID 26186941.
- ↑ 114.0 114.1 "Oxidative DNA Damage Modulates DNA Methylation Pattern in Human Breast Cancer 1 (BRCA1) Gene via the Crosstalk between DNA Polymerase β and a de novo DNA Methyltransferase". Cells 9 (1): 225. January 2020. doi:10.3390/cells9010225. PMID 31963223.
- ↑ "Recruitment of DNA methyltransferase I to DNA repair sites". Proc Natl Acad Sci U S A 102 (25): 8905–9. June 2005. doi:10.1073/pnas.0501034102. PMID 15956212. Bibcode: 2005PNAS..102.8905M.
- ↑ 116.0 116.1 "DNA damage, homology-directed repair, and DNA methylation". PLOS Genet 3 (7): e110. July 2007. doi:10.1371/journal.pgen.0030110. PMID 17616978.
- ↑ 117.0 117.1 "Double strand breaks can initiate gene silencing and SIRT1-dependent onset of DNA methylation in an exogenous promoter CpG island". PLOS Genet 4 (8): e1000155. August 2008. doi:10.1371/journal.pgen.1000155. PMID 18704159.
- ↑ "Rapid and transient recruitment of DNMT1 to DNA double-strand breaks is mediated by its interaction with multiple components of the DNA damage response machinery". Hum Mol Genet 20 (1): 126–40. January 2011. doi:10.1093/hmg/ddq451. PMID 20940144.
- ↑ "DNA damage and Repair Modify DNA methylation and Chromatin Domain of the Targeted Locus: Mechanism of allele methylation polymorphism". Sci Rep 6: 33222. September 2016. doi:10.1038/srep33222. PMID 27629060. Bibcode: 2016NatSR...633222R.
- ↑ "Detection of CRISPR-mediated genome modifications through altered methylation patterns of CpG islands". BMC Genomics 21 (1): 856. December 2020. doi:10.1186/s12864-020-07233-2. PMID 33267773.
- ↑ "Non-homologous end joining induced alterations in DNA methylation: A source of permanent epigenetic change". Oncotarget 8 (25): 40359–40372. June 2017. doi:10.18632/oncotarget.16122. PMID 28423717.
- ↑ 122.0 122.1 "Epigenetic research in cancer epidemiology: trends, opportunities, and challenges". Cancer Epidemiology, Biomarkers & Prevention 23 (2): 223–33. February 2014. doi:10.1158/1055-9965.EPI-13-0573. PMID 24326628.
- ↑ 123.0 123.1 "Studying epigenetics using ChIP". Abcam. https://www.abcam.com/epigenetics/studying-epigenetics-using-chip.
- ↑ 124.0 124.1 "Combined Immunofluorescence, RNA Fluorescent in Situ Hybridization, and DNA Fluorescent in Situ Hybridization to Study Chromatin Changes, Transcriptional Activity, Nuclear Organization, and X-Chromosome Inactivation". The Nucleus. Methods in Molecular Biology. 463. Clifton, N.J.. 2008. pp. 297–308. doi:10.1007/978-1-59745-406-3_18. ISBN 978-1-58829-977-2.
- ↑ 125.0 125.1 "Fluorescence in situ hybridization (FISH).". Nature Education 1 (1): 171. 2008. https://www.nature.com/scitable/topicpage/fluorescence-in-situ-hybridization-fish-327/.
- ↑ 126.0 126.1 126.2 126.3 "Improved quantification of DNA methylation using methylation-sensitive restriction enzymes and real-time PCR". Epigenetics 2 (2): 86–91. 2007. doi:10.4161/epi.2.2.4203. PMID 17965602.
- ↑ Simpson, Jared T.; Workman, Rachael E.; Zuzarte, P. C.; David, Matei; Dursi, L. J.; Timp, Winston (2017). "Detecting DNA cytosine methylation using nanopore sequencing" (in en). Nature Methods 14 (4): 407–410. doi:10.1038/nmeth.4184. ISSN 1548-7105. PMID 28218898. https://www.nature.com/articles/nmeth.4184.
- ↑ "Concepts of Organization the Leverage of Ciliate Protozoa". A Conceptual History of Modern Embryology. Developmental Biology. 7. 1991. pp. 229–258. doi:10.1007/978-1-4615-6823-0_11. ISBN 978-1-4615-6825-4.
- ↑ Genesis: the evolution of biology. Oxford: Oxford University Press. 2003. ISBN 978-0-19-515619-5. https://archive.org/details/genesisevolution00sapp.
- ↑ Cycles of Contingency: Developmental Systems and Evolution (Life and Mind: Philosophical Issues in Biology and Psychology). Cambridge, Massachusetts: The MIT Press. 2003. ISBN 978-0-262-65063-2.
- ↑ "Tissue-specific profiling reveals distinctive regulatory architectures for ubiquitous, germline and somatic genes". bioRxiv: 2020.02.20.958579. 2020-02-20. doi:10.1101/2020.02.20.958579.
- ↑ 132.0 132.1 "Nucleosome repositioning links DNA (de)methylation and differential CTCF binding during stem cell development". Genome Research 24 (8): 1285–95. August 2014. doi:10.1101/gr.164418.113. PMID 24812327.
- ↑ "Variants of core histones and their roles in cell fate decisions, development and cancer". Nature Reviews. Molecular Cell Biology 18 (5): 299–314. May 2017. doi:10.1038/nrm.2016.166. PMID 28144029. https://www.nature.com/articles/nrm.2016.166.
- ↑ "Histone H3.3 maintains genome integrity during mammalian development". Genes & Development 29 (13): 1377–92. July 2015. doi:10.1101/gad.264150.115. PMID 26159997.
- ↑ "The 3D genome" (in en). 2 September 2019. https://www.nature.com/collections/rsxlmsyslk/.
- ↑ "Engrams and circuits crucial for systems consolidation of a memory". Science 356 (6333): 73–78. April 2017. doi:10.1126/science.aam6808. PMID 28386011. Bibcode: 2017Sci...356...73K.
- ↑ 137.0 137.1 "Profiling DNA break sites and transcriptional changes in response to contextual fear learning". PLOS ONE 16 (7): e0249691. 2021. doi:10.1371/journal.pone.0249691. PMID 34197463. Bibcode: 2021PLoSO..1649691S.
- ↑ "Real-time visualization of mRNA synthesis during memory formation in live mice". Proc Natl Acad Sci U S A 119 (27): e2117076119. July 2022. doi:10.1073/pnas.2117076119. PMID 35776545. Bibcode: 2022PNAS..11917076L.
- ↑ "Activation of immediate early genes and memory formation". Cell Mol Life Sci 55 (4): 564–74. April 1999. doi:10.1007/s000180050315. PMID 10357227.
- ↑ 140.0 140.1 "Rescue of aging-associated decline in Dnmt3a2 expression restores cognitive abilities". Nat Neurosci 15 (8): 1111–3. July 2012. doi:10.1038/nn.3151. PMID 22751036.
- ↑ 141.0 141.1 "EGR1 recruits TET1 to shape the brain methylome during development and upon neuronal activity". Nat Commun 10 (1): 3892. August 2019. doi:10.1038/s41467-019-11905-3. PMID 31467272. Bibcode: 2019NatCo..10.3892S.
- ↑ "Isoform-specific localization of DNMT3A regulates DNA methylation fidelity at bivalent CpG islands". EMBO J 36 (23): 3421–3434. December 2017. doi:10.15252/embj.201797038. PMID 29074627.
- ↑ "Fear memory formation can affect a different memory: fear conditioning affects the extinction, but not retrieval, of conditioned taste aversion (CTA) memory". Front Behav Neurosci 8: 324. 2014. doi:10.3389/fnbeh.2014.00324. PMID 25324744.
- ↑ "DNA methylation and its basic function". Neuropsychopharmacology 38 (1): 23–38. January 2013. doi:10.1038/npp.2012.112. PMID 22781841.
- ↑ 145.0 145.1 "DNA methylation changes in plasticity genes accompany the formation and maintenance of memory". Nat Neurosci 19 (1): 102–10. January 2016. doi:10.1038/nn.4194. PMID 26656643.
- ↑ "The involvement of the anterior cingulate cortex in remote contextual fear memory". Science 304 (5672): 881–3. May 2004. doi:10.1126/science.1094804. PMID 15131309. Bibcode: 2004Sci...304..881F.
- ↑ "Aging in the Brain: New Roles of Epigenetics in Cognitive Decline". The Neuroscientist 24 (5): 516–525. October 2018. doi:10.1177/1073858418780971. PMID 29877135.
- ↑ "Epigenetic mechanisms related to cognitive decline during aging". Journal of Neuroscience Research 98 (2): 234–246. February 2020. doi:10.1002/jnr.24436. PMID 31045277.
- ↑ "Epigenetic changes during ageing and their underlying mechanisms". Biogerontology 21 (4): 423–443. August 2020. doi:10.1007/s10522-020-09874-y. PMID 32356238.
- ↑ "The ageing epigenome and its rejuvenation". Nature Reviews. Molecular Cell Biology 21 (3): 137–150. March 2020. doi:10.1038/s41580-019-0204-5. PMID 32020082.
- ↑ "Cellular reprogramming and epigenetic rejuvenation". Clinical Epigenetics 13 (1): 170. September 2021. doi:10.1186/s13148-021-01158-7. PMID 34488874.
- ↑ "The emerging field of epigenetics in neurodegeneration and neuroprotection". Nature Reviews. Neuroscience 18 (6): 347–361. May 2017. doi:10.1038/nrn.2017.46. PMID 28515491.
- ↑ "Epigenetic regulation of cognition: A circumscribed review of the field". Development and Psychopathology 28 (4pt2): 1285–1304. November 2016. doi:10.1017/S0954579416000857. PMID 27691982.
- ↑ "Epigenetics of the developing and aging brain: Mechanisms that regulate onset and outcomes of brain reorganization". Neuroscience and Biobehavioral Reviews 125: 503–516. June 2021. doi:10.1016/j.neubiorev.2021.02.040. PMID 33657435.
- ↑ "Epigenetics, estrogenic endocrine-disrupting chemicals (EDCs), and the brain". Endocrine-Disrupting Chemicals. Advances in Pharmacology. 92. 2021. pp. 73–99. doi:10.1016/bs.apha.2021.03.006. ISBN 9780128234662.
- ↑ "Choline, the brain and neurodegeneration: insights from epigenetics". Frontiers in Bioscience 23 (6): 1113–1143. January 2018. doi:10.2741/4636. PMID 28930592.
- ↑ "Brain foods - the role of diet in brain performance and health". Nutrition Reviews 79 (6): 693–708. May 2021. doi:10.1093/nutrit/nuaa091. PMID 32989449.
- ↑ "Physical exercise as an epigenetic modulator of brain plasticity and cognition". Neuroscience and Biobehavioral Reviews 80: 443–456. September 2017. doi:10.1016/j.neubiorev.2017.06.012. PMID 28666827.
- ↑ "New discovery: Synapse hiding in the mice brain may advance our understanding of neuronal communication". interestingengineering.com. 4 September 2022. https://interestingengineering.com/science/new-discovery-synapse-hiding-in-mice-brain.
- ↑ "A serotonergic axon-cilium synapse drives nuclear signaling to alter chromatin accessibility" (in English). Cell 185 (18): 3390–3407.e18. September 2022. doi:10.1016/j.cell.2022.07.026. PMID 36055200.
- University press release: "Scientists discover new kind of synapse in neurons' tiny hairs" (in en). Howard Hughes Medical Institute via phys.org. https://phys.org/news/2022-09-scientists-kind-synapse-neurons-tiny.html.
- ↑ "Epigenetics and brain evolution". Epigenomics 3 (2): 183–191. April 2011. doi:10.2217/epi.11.10. PMID 22122280.
- ↑ "Critical Notice: Cycles of Contingency – Developmental Systems and Evolution". Biology & Philosophy 20 (2–3): 517–44. March 2005. doi:10.1007/s10539-004-0836-4.
- ↑ Chapter: "Nervous System Development" in "Epigenetics," by Benedikt Hallgrimsson and Brian Hall
- ↑ "'Open minded' cells: how cells can change fate". Trends in Cell Biology 17 (3): 101–6. March 2007. doi:10.1016/j.tcb.2006.12.005. PMID 17194589. http://cromatina.icb.ufmg.br/biomol/seminarios/outros/grupo_open.pdf. "This might suggest that plant cells do not use or require a cellular memory mechanism and just respond to positional information. However, it has been shown that plants do use cellular memory mechanisms mediated by PcG proteins in several processes, ... (p. 104)".
- ↑ "Maternal methyl supplements in mice affect epigenetic variation and DNA methylation of offspring". The Journal of Nutrition 132 (8 Suppl): 2393S–2400S. August 2002. doi:10.1093/jn/132.8.2393S. PMID 12163699.
- ↑ "Transposable elements: targets for early nutritional effects on epigenetic gene regulation". Molecular and Cellular Biology 23 (15): 5293–300. August 2003. doi:10.1128/MCB.23.15.5293-5300.2003. PMID 12861015.
- ↑ "The agouti mouse model: an epigenetic biosensor for nutritional and environmental alterations on the fetal epigenome". Nutrition Reviews 66 (Suppl 1): S7-11. August 2008. doi:10.1111/j.1753-4887.2008.00056.x. PMID 18673496.
- ↑ "Aberrant DNA methylation as a diagnostic biomarker of diabetic embryopathy". Genetics in Medicine 21 (11): 2453–2461. November 2019. doi:10.1038/s41436-019-0516-z. PMID 30992551.
- ↑ "Fearful Memories Passed Down to Mouse Descendants: Genetic imprint from traumatic experiences carries through at least two generations". Nature Magazine. 1 December 2013. https://www.scientificamerican.com/article/fearful-memories-passed-down/.
- ↑ "Mice can 'warn' sons, grandsons of dangers via sperm". 13 December 2013. http://medicalxpress.com/news/2013-12-mice-sons-grandsons-dangers-sperm.html#ajTabs.
- ↑ "Too much success for recent groundbreaking epigenetic experiments". Genetics 198 (2): 449–451. October 2014. doi:10.1534/genetics.114.163998. PMID 25316784.
- ↑ "Parental olfactory experience influences behavior and neural structure in subsequent generations". Nature Neuroscience 17 (1): 89–96. January 2014. doi:10.1038/nn.3594. PMID 24292232. (see comment by Gonzalo Otazu)
- ↑ "Epigenetics Paper Raises Questions". http://www.the-scientist.com/?articles.view/articleNo/41239/title/Epigenetics-Paper-Raises-Questions/.
- ↑ Hoekstra RF (2000). Evolution: an introduction. Oxford: Oxford University Press. p. 285. ISBN 978-0-19-854968-0.
- ↑ Evolution in four dimensions: genetic, epigenetic, behavioral, and symbolic variation in the history of life. Cambridge, Massachusetts: MIT Press. 2005. ISBN 978-0-262-10107-3.
- ↑ See also Denis Noble: The Music of Life, esp pp. 93–98 and p. 48, where he cites Jablonka & Lamb and Massimo Pigliucci's review of Jablonka and Lamb in Nature 435, 565–566 (2 June 2005)
- ↑ "Beyond DNA: integrating inclusive inheritance into an extended theory of evolution". Nature Reviews. Genetics 12 (7): 475–86. June 2011. doi:10.1038/nrg3028. PMID 21681209.
- ↑ "Models of a dual inheritance system". Journal of Theoretical Biology 143 (1): 41–53. March 1990. doi:10.1016/S0022-5193(05)80287-5. PMID 2359317. Bibcode: 1990JThBi.143...41M.
- ↑ "The frailty of adaptive hypotheses for the origins of organismal complexity". Proceedings of the National Academy of Sciences of the United States of America 104 (Suppl 1): 8597–604. May 2007. doi:10.1073/pnas.0702207104. PMID 17494740. Bibcode: 2007PNAS..104.8597L.
- ↑ "The extended evolutionary synthesis and the role of soft inheritance in evolution". Proceedings. Biological Sciences 279 (1740): 2913–21. August 2012. doi:10.1098/rspb.2012.0273. PMID 22593110.
- ↑ "Timescales of genetic and epigenetic inheritance". Cell 128 (4): 655–68. February 2007. doi:10.1016/j.cell.2007.01.023. PMID 17320504.
- ↑ "The evolution of reversible switches in the presence of irreversible mimics". Evolution; International Journal of Organic Evolution 63 (9): 2350–62. September 2009. doi:10.1111/j.1558-5646.2009.00729.x. PMID 19486147.
- ↑ "Rate, spectrum, and evolutionary dynamics of spontaneous epimutations". Proceedings of the National Academy of Sciences of the United States of America 112 (21): 6676–81. May 2015. doi:10.1073/pnas.1424254112. PMID 25964364. Bibcode: 2015PNAS..112.6676V.
- ↑ "Complex adaptations can drive the evolution of the capacitor [PSI, even with realistic rates of yeast sex"]. PLOS Genetics 5 (6): e1000517. June 2009. doi:10.1371/journal.pgen.1000517. PMID 19521499.
- ↑ "Transgenerational epigenetic inheritance: prevalence, mechanisms, and implications for the study of heredity and evolution". The Quarterly Review of Biology 84 (2): 131–76. June 2009. doi:10.1086/598822. PMID 19606595. http://compgen.unc.edu/wiki/images/d/df/JablonkaQtrRevBio2009.pdf. Retrieved 1 November 2017.
- ↑ Davies, Hazel (2008). Do Butterflies Bite?: Fascinating Answers to Questions about Butterflies and Moths (Animals Q&A). Rutgers University Press.
- ↑ "Relics of repeat-induced point mutation direct heterochromatin formation in Neurospora crassa". Genome Research 19 (3): 427–37. March 2009. doi:10.1101/gr.086231.108. PMID 19092133.
- ↑ 188.0 188.1 Epigenetics. Norfolk, England: Caister Academic Press. 2008. ISBN 978-1-904455-23-3.
- ↑ "Modeling kinetic rate variation in third generation DNA sequencing data to detect putative modifications to DNA bases". Genome Research 23 (1): 129–41. January 2013. doi:10.1101/gr.136739.111. PMID 23093720.
- ↑ "Entering the era of bacterial epigenomics with single molecule real time DNA sequencing". Current Opinion in Microbiology 16 (2): 192–8. April 2013. doi:10.1016/j.mib.2013.01.011. PMID 23434113.
- ↑ "Comprehensive methylome characterization of Mycoplasma genitalium and Mycoplasma pneumoniae at single-base resolution". PLOS Genetics 9 (1): e1003191. 2013. doi:10.1371/journal.pgen.1003191. PMID 23300489.
- ↑ "The methylomes of six bacteria". Nucleic Acids Research 40 (22): 11450–62. December 2012. doi:10.1093/nar/gks891. PMID 23034806.
- ↑ "Genome-wide mapping of methylated adenine residues in pathogenic Escherichia coli using single-molecule real-time sequencing". Nature Biotechnology 30 (12): 1232–9. December 2012. doi:10.1038/nbt.2432. PMID 23138224.
- ↑ "Bacterial Epigenomics: Coming of Age". mSystems 6 (4): e0074721. August 2021. doi:10.1128/mSystems.00747-21. PMID 34402642.
- ↑ "Epigenetic gene regulation in the bacterial world". Microbiology and Molecular Biology Reviews 70 (3): 830–56. September 2006. doi:10.1128/MMBR.00016-06. PMID 16959970.
- ↑ "A random six-phase switch regulates pneumococcal virulence via global epigenetic changes". Nature Communications 5: 5055. September 2014. doi:10.1038/ncomms6055. PMID 25268848. Bibcode: 2014NatCo...5.5055M.
- ↑ "Epigenomic characterization of Clostridioides difficile finds a conserved DNA methyltransferase that mediates sporulation and pathogenesis". Nature Microbiology 5 (1): 166–180. January 2020. doi:10.1038/s41564-019-0613-4. PMID 31768029.
- ↑ "The multidimensional nature of epigenetic information and its role in disease". Discovery Medicine 11 (58): 233–43. March 2011. PMID 21447282.
- ↑ "Medicine's New Epicenter? Epigenetics: New field of epigenetics may hold the secret to flipping cancer's "off" switch.". CURE (Cancer Updates, Research and Education). Winter 2008. http://www.curetoday.com/index.cfm/fuseaction/article.show/id/2/article_id/949.
- ↑ 200.0 200.1 200.2 "Epigenetic differences arise during the lifetime of monozygotic twins". Proceedings of the National Academy of Sciences of the United States of America 102 (30): 10604–9. July 2005. doi:10.1073/pnas.0500398102. PMID 16009939. Bibcode: 2005PNAS..10210604F.
- ↑ 201.0 201.1 "DNA methylation profiles in monozygotic and dizygotic twins". Nature Genetics 41 (2): 240–5. February 2009. doi:10.1038/ng.286. PMID 19151718.
- ↑ "The Claim: Identical Twins Have Identical DNA". New York Times. 11 March 2008. https://www.nytimes.com/2008/03/11/health/11real.html.
- ↑ "Epigenetics lessons from twins: prospects for autoimmune disease". Clinical Reviews in Allergy & Immunology 39 (1): 30–41. August 2010. doi:10.1007/s12016-009-8168-4. PMID 19653134.
- ↑ "Potential Diagnostic and Prognostic Biomarkers of Epigenetic Drift within the Cardiovascular Compartment". BioMed Research International 2016: 2465763. 2016. doi:10.1155/2016/2465763. PMID 26942189.
- ↑ Online Mendelian Inheritance in Man (OMIM) 105830
- ↑ "Genomic imprinting in mammals: emerging themes and established theories". PLOS Genetics 2 (11): e147. November 2006. doi:10.1371/journal.pgen.0020147. PMID 17121465.
- ↑ "Angelman and Prader–Willi syndromes share a common chromosome 15 deletion but differ in parental origin of the deletion". American Journal of Medical Genetics 32 (2): 285–90. February 1989. doi:10.1002/ajmg.1320320235. PMID 2564739.
- ↑ "Neural stem cells from a mouse model of Rett syndrome are prone to senescence, show reduced capacity to cope with genotoxic stress, and are impaired in the differentiation process". Experimental & Molecular Medicine 50 (3): 1. March 2018. doi:10.1038/s12276-017-0005-x. PMID 29563495.
- ↑ A person's paternal grandson is the son of a son of that person; a maternal grandson is the son of a daughter.
- ↑ "Sex-specific, male-line transgenerational responses in humans". European Journal of Human Genetics 14 (2): 159–66. February 2006. doi:10.1038/sj.ejhg.5201538. PMID 16391557. Robert Winston refers to this study in a "Lecture". http://www.dundee.ac.uk/externalrelations/events/lectures.html.
- ↑ "NOVA | Transcripts | Ghost in Your Genes". PBS. 16 October 2007. https://www.pbs.org/wgbh/nova/transcripts/3413_genes.html.
- ↑ "Epigenetic modulation of macrophage polarization- perspectives in diabetic wounds". Journal of Diabetes and Its Complications 32 (5): 524–530. May 2018. doi:10.1016/j.jdiacomp.2018.01.015. PMID 29530315.
- ↑ "Off-Target drug effects resulting in altered gene expression events with epigenetic and "Quasi-Epigenetic" origins". Pharmacological Research 107: 229–233. May 2016. doi:10.1016/j.phrs.2016.03.028. PMID 27025785.
- ↑ "Influence of life stress on depression: moderation by a polymorphism in the 5-HTT gene". Science 301 (5631): 386–9. July 2003. doi:10.1126/science.1083968. PMID 12869766. Bibcode: 2003Sci...301..386C.
- ↑ "Understanding Epigenetic Effects of Endocrine Disrupting Chemicals: From Mechanisms to Novel Test Methods". Basic & Clinical Pharmacology & Toxicology 122 (1): 38–45. January 2018. doi:10.1111/bcpt.12878. PMID 28842957.
- ↑ "Persistence of Early-Life Stress on the Epigenome: Nonhuman Primate Observations☆". Reference Module in Neuroscience and Biobehavioral Psychology. 2017. doi:10.1016/B978-0-12-809324-5.02862-5. ISBN 9780128093245.
- ↑ 217.0 217.1 Behavioral Genetics (Seventh ed.). Worth Publishers. 2017. pp. 152–153. ISBN 978-1-4292-4215-8. https://books.google.com/books?id=OytMMAEACAAJ.
- ↑ "Transgenerational epigenetic inheritance: myths and mechanisms". Cell 157 (1): 95–109. March 2014. doi:10.1016/j.cell.2014.02.045. PMID 24679529.
- ↑ "Transcriptional and epigenetic mechanisms of addiction". Nature Reviews. Neuroscience 12 (11): 623–37. October 2011. doi:10.1038/nrn3111. PMID 21989194.
- ↑ "Cellular basis of memory for addiction". Dialogues in Clinical Neuroscience 15 (4): 431–43. December 2013. doi:10.31887/DCNS.2013.15.4/enestler. PMID 24459410.
- ↑ "Molecular neurobiology of addiction: what's all the (Δ)FosB about?". The American Journal of Drug and Alcohol Abuse 40 (6): 428–37. November 2014. doi:10.3109/00952990.2014.933840. PMID 25083822. "Conclusions
ΔFosB is an essential transcription factor implicated in the molecular and behavioral pathways of addiction following repeated drug exposure. The formation of ΔFosB in multiple brain regions, and the molecular pathway leading to the formation of AP-1 complexes is well understood. The establishment of a functional purpose for ΔFosB has allowed further determination as to some of the key aspects of its molecular cascades, involving effectors such as GluR2 (87,88), Cdk5 (93) and NFkB (100). Moreover, many of these molecular changes identified are now directly linked to the structural, physiological and behavioral changes observed following chronic drug exposure (60,95,97,102). New frontiers of research investigating the molecular roles of ΔFosB have been opened by epigenetic studies, and recent advances have illustrated the role of ΔFosB acting on DNA and histones, truly as a molecular switch (34). As a consequence of our improved understanding of ΔFosB in addiction, it is possible to evaluate the addictive potential of current medications (119), as well as use it as a biomarker for assessing the efficacy of therapeutic interventions (121,122,124). Some of these proposed interventions have limitations (125) or are in their infancy (75). However, it is hoped that some of these preliminary findings may lead to innovative treatments, which are much needed in addiction.". - ↑ "Epigenetic regulation in drug addiction". Annals of Agricultural and Environmental Medicine 19 (3): 491–6. 2012. PMID 23020045. "For these reasons, ΔFosB is considered a primary and causative transcription factor in creating new neural connections in the reward centre, prefrontal cortex, and other regions of the limbic system. This is reflected in the increased, stable and long-lasting level of sensitivity to cocaine and other drugs, and tendency to relapse even after long periods of abstinence. These newly constructed networks function very efficiently via new pathways as soon as drugs of abuse are further taken ... In this way, the induction of CDK5 gene expression occurs together with suppression of the G9A gene coding for dimethyltransferase acting on the histone H3. A feedback mechanism can be observed in the regulation of these 2 crucial factors that determine the adaptive epigenetic response to cocaine. This depends on ΔFosB inhibiting G9a gene expression, i.e. H3K9me2 synthesis which in turn inhibits transcription factors for ΔFosB. For this reason, the observed hyper-expression of G9a, which ensures high levels of the dimethylated form of histone H3, eliminates the neuronal structural and plasticity effects caused by cocaine by means of this feedback which blocks ΔFosB transcription".
- ↑ "Mechanisms of transgenerational inheritance of addictive-like behaviors". Neuroscience 264: 198–206. April 2014. doi:10.1016/j.neuroscience.2013.07.064. PMID 23920159.
- ↑ "Transgenerational Inheritance of Paternal Neurobehavioral Phenotypes: Stress, Addiction, Ageing and Metabolism". Molecular Neurobiology 53 (9): 6367–6376. November 2016. doi:10.1007/s12035-015-9526-2. PMID 26572641.
- ↑ "Elevated paternal glucocorticoid exposure alters the small noncoding RNA profile in sperm and modifies anxiety and depressive phenotypes in the offspring". Translational Psychiatry 6 (6): e837. June 2016. doi:10.1038/tp.2016.109. PMID 27300263.
- ↑ Kee, J., Thudium, S., Renner, D.M. et al. (05 October 2022) "SARS-CoV-2 disrupts host epigenetic regulation via histone mimicry". Nature PMCID: PMC9533993 DOI: 10.1038/s41586-022-05282-z (2022). Retrieved 7 October 2022.
- ↑ "Crosstalk between genetic and epigenetic information through cytosine deamination". Trends in Genetics 26 (10): 443–8. October 2010. doi:10.1016/j.tig.2010.07.005. PMID 20800313.
- ↑ "Nonantibiotic Effects of Fluoroquinolones in Mammalian Cells". The Journal of Biological Chemistry 290 (36): 22287–97. September 2015. doi:10.1074/jbc.M115.671222. PMID 26205818.
- ↑ "The histone methyltransferase inhibitor BIX01294 enhances the cardiac potential of bone marrow cells". Stem Cells and Development 22 (4): 654–67. February 2013. doi:10.1089/scd.2012.0181. PMID 22994322.
- ↑ "Inhibition of G9a Histone Methyltransferase Converts Bone Marrow Mesenchymal Stem Cells to Cardiac Competent Progenitors". Stem Cells International 2015: 270428. 2015. doi:10.1155/2015/270428. PMID 26089912.
- ↑ "CD44 regulates epigenetic plasticity by mediating iron endocytosis". Nature Chemistry 12 (10): 929–938. October 2020. doi:10.1038/s41557-020-0513-5. PMID 32747755. Bibcode: 2020NatCh..12..929M.
- ↑ "A druggable copper-signalling pathway that drives inflammation". Nature 617 (7960): 386–394. May 2023. doi:10.1038/s41586-023-06017-4. PMID 37100912. Bibcode: 2023Natur.617..386S.
- ↑ "RNA epigenetics". Translational Research 165 (1): 28–35. January 2015. doi:10.1016/j.trsl.2014.04.003. PMID 24768686.
- ↑ "Epigenetics: Roles and therapeutic implications of non-coding RNA modifications in human cancers". Molecular Therapy. Nucleic Acids 25: 67–82. September 2021. doi:10.1016/j.omtn.2021.04.021. PMID 34188972.
- ↑ "Epigenome editing: targeted manipulation of epigenetic modifications in plants". Genes & Genomics 44 (3): 307–315. March 2022. doi:10.1007/s13258-021-01199-5. PMID 35000141.
- ↑ 236.0 236.1 236.2 236.3 236.4 236.5 236.6 "Genetic variation influencing DNA methylation provides insights into molecular mechanisms regulating genomic function". Nature Genetics 54 (1): 18–29. January 2022. doi:10.1038/s41588-021-00969-x. PMID 34980917. https://push-zb.helmholtz-muenchen.de/frontdoor.php?source_opus=64018. Retrieved 20 January 2023.
- ↑ "Protein tyrosine phosphatase UBASH3B is overexpressed in triple-negative breast cancer and promotes invasion and metastasis". Proceedings of the National Academy of Sciences of the United States of America 110 (27): 11121–11126. July 2013. doi:10.1073/pnas.1300873110. PMID 23784775. Bibcode: 2013PNAS..11011121L.
- ↑ "Meta-analysis of genome-wide association studies for height and body mass index in ~700000 individuals of European ancestry". Human Molecular Genetics 27 (20): 3641–3649. October 2018. doi:10.1093/hmg/ddy271. PMID 30124842.
- ↑ "Meta-analysis of genome-wide association studies for body fat distribution in 694 649 individuals of European ancestry". Human Molecular Genetics 28 (1): 166–174. January 2019. doi:10.1093/hmg/ddy327. PMID 30239722.
- ↑ "Shared genetic and experimental links between obesity-related traits and asthma subtypes in UK Biobank". The Journal of Allergy and Clinical Immunology 145 (2): 537–549. February 2020. doi:10.1016/j.jaci.2019.09.035. PMID 31669095.
- ↑ "Evaluating the relationship between circulating lipoprotein lipids and apolipoproteins with risk of coronary heart disease: A multivariable Mendelian randomisation analysis". PLOS Medicine 17 (3): e1003062. March 2020. doi:10.1371/journal.pmed.1003062. PMID 32203549.
- ↑ "Blood cell transcriptomic-based early biomarkers of adverse programming effects of gestational calorie restriction and their reversibility by leptin supplementation". Scientific Reports 5 (1): 9088. March 2015. doi:10.1038/srep09088. PMID 25766068. Bibcode: 2015NatSR...5E9088K.
- ↑ "From the era of genome analysis to the era of genomic drug discovery: a pioneering example of rheumatoid arthritis". Clinical Genetics 86 (5): 432–440. November 2014. doi:10.1111/cge.12465. PMID 25060537.
- ↑ "FADS1-FADS2 genetic polymorphisms are associated with fatty acid metabolism through changes in DNA methylation and gene expression". Clinical Epigenetics 10 (1): 113. August 2018. doi:10.1186/s13148-018-0545-5. PMID 30157936.
- ↑ "Genome-wide association study of plasma N6 polyunsaturated fatty acids within the cohorts for heart and aging research in genomic epidemiology consortium". Circulation: Cardiovascular Genetics 7 (3): 321–331. June 2014. doi:10.1161/circgenetics.113.000208. PMID 24823311.
- ↑ "An atlas of genetic influences on human blood metabolites". Nature Genetics 46 (6): 543–550. June 2014. doi:10.1038/ng.2982. PMID 24816252.
- ↑ "A GWAS of 170,000 Individuals Identifies Thousands of Alleles Perturbing Blood Cell Traits, Many of Which Are in Super Enhancers Setting Cell Identity". Blood 128 (22): 2652. 2016-12-02. doi:10.1182/blood.v128.22.2652.2652. ISSN 0006-4971.
- ↑ "PhenoScanner V2: an expanded tool for searching human genotype-phenotype associations". Bioinformatics 35 (22): 4851–4853. November 2019. doi:10.1093/bioinformatics/btz469. PMID 31233103.
- ↑ "Epigenetics: It doesn't mean what quacks think it means". Science-Based Medicine. 4 February 2013. https://www.sciencebasedmedicine.org/epigenetics-it-doesnt-mean-what-quacks-think-it-means/.
Lua error: Internal error: The interpreter exited with status 1.
Further reading
- "Not really identical: epigenetic differences in monozygotic twins and implications for twin studies in psychiatry". American Journal of Medical Genetics. Part C, Seminars in Medical Genetics 151C (2): 136–41. May 2009. doi:10.1002/ajmg.c.30206. PMID 19378334.
- "What is Epigenetics?". 15 Aug 2022. https://www.cdc.gov/genomics/disease/epigenetics.htm.
External links
Lua error: Internal error: The interpreter exited with status 1.
- "Epigenetics & Inheritance". https://learn.genetics.utah.edu/content/epigenetics/inheritance/.
- The Human Epigenome Project (HEP)
- The Epigenome Network of Excellence (NoE)
- Canadian Epigenetics, Environment and Health Research Consortium (CEEHRC)
- The Epigenome Network of Excellence (NoE) – public international site
- "DNA Is Not Destiny" – Discover magazine cover story
- "The Ghost In Your Genes", Horizon (2005), BBC
- Epigenetics article at Hopkins Medicine
- Towards a global map of epigenetic variation
Lua error: Internal error: The interpreter exited with status 1. Lua error: Internal error: The interpreter exited with status 1. Lua error: Internal error: The interpreter exited with status 1. Lua error: Internal error: The interpreter exited with status 1. Lua error: Internal error: The interpreter exited with status 1.
Lua error: Internal error: The interpreter exited with status 1.