Biology:Cancer epigenetics
Cancer epigenetics is the study of epigenetic modifications to the DNA of cancer cells that do not involve a change in the nucleotide sequence, but instead involve a change in the way the genetic code is expressed. Epigenetic mechanisms are necessary to maintain normal sequences of tissue specific gene expression and are crucial for normal development.[1] They may be just as important, if not even more important, than genetic mutations in a cell's transformation to cancer. The disturbance of epigenetic processes in cancers, can lead to a loss of expression of genes that occurs about 10 times more frequently by transcription silencing (caused by epigenetic promoter hypermethylation of CpG islands) than by mutations. As Vogelstein et al. points out, in a colorectal cancer there are usually about 3 to 6 driver mutations and 33 to 66 hitchhiker or passenger mutations.[2] However, in colon tumors compared to adjacent normal-appearing colonic mucosa, there are about 600 to 800 heavily methylated CpG islands in the promoters of genes in the tumors while these CpG islands are not methylated in the adjacent mucosa.[3][4][5] Manipulation of epigenetic alterations holds great promise for cancer prevention, detection, and therapy.[6][7] In different types of cancer, a variety of epigenetic mechanisms can be perturbed, such as the silencing of tumor suppressor genes and activation of oncogenes by altered CpG island methylation patterns, histone modifications, and dysregulation of DNA binding proteins. There are several medications which have epigenetic impact, that are now used in a number of these diseases.
Mechanisms
DNA methylation
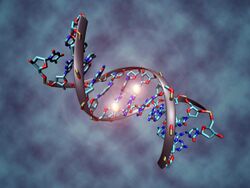
In somatic cells, patterns of DNA methylation are in general transmitted to daughter cells with high fidelity.[8] Typically, this methylation only occurs at cytosines that are located 5' to guanosine in the CpG dinucleotides of higher order eukaryotes.[9] However, epigenetic DNA methylation differs between normal cells and tumor cells in humans. The "normal" CpG methylation profile is often inverted in cells that become tumorigenic.[10] In normal cells, CpG islands preceding gene promoters are generally unmethylated, and tend to be transcriptionally active, while other individual CpG dinucleotides throughout the genome tend to be methylated. However, in cancer cells, CpG islands preceding tumor suppressor gene promoters are often hypermethylated, while CpG methylation of oncogene promoter regions and parasitic repeat sequences is often decreased.[11]
Hypermethylation of tumor suppressor gene promoter regions can result in silencing of those genes. This type of epigenetic mutation allows cells to grow and reproduce uncontrollably, leading to tumorigenesis.[10] The addition of methyl groups to cytosines causes the DNA to coil tightly around the histone proteins, resulting in DNA that can not undergo transcription (transcriptionally silenced DNA). Genes commonly found to be transcriptionally silenced due to promoter hypermethylation include: Cyclin-dependent kinase inhibitor p16, a cell-cycle inhibitor; MGMT, a DNA repair gene; APC, a cell cycle regulator; MLH1, a DNA-repair gene; and BRCA1, another DNA-repair gene.[10][12] Indeed, cancer cells can become addicted to the transcriptional silencing, due to promoter hypermethylation, of some key tumor suppressor genes, a process known as epigenetic addiction.[13]
Hypomethylation of CpG dinucleotides in other parts of the genome leads to chromosome instability due to mechanisms such as loss of imprinting and reactivation of transposable elements.[14][15][16][17] Loss of imprinting of insulin-like growth factor gene (IGF2) increases risk of colorectal cancer and is associated with Beckwith-Wiedemann syndrome which significantly increases the risk of cancer for newborns.[18] In healthy cells, CpG dinucleotides of lower densities are found within coding and non-coding intergenic regions. Expression of some repetitive sequences and meiotic recombination at centromeres are repressed through methylation [19]
The entire genome of a cancerous cell contains significantly less methylcytosine than the genome of a healthy cell. In fact, cancer cell genomes have 20-50% less methylation at individual CpG dinucleotides across the genome.[14][15][16][17] CpG islands found in promoter regions are usually protected from DNA methylation. In cancer cells CpG islands are hypomethylated [20] The regions flanking CpG islands called CpG island shores are where most DNA methylation occurs in the CpG dinucleotide context. Cancer cells are deferentially methylated at CpG island shores. In cancer cells, hypermethylation in the CpG island shores move into CpG islands, or hypomethylation of CpG islands move into CpG island shores eliminating sharp epigenetic boundaries between these genetic elements.[21] In cancer cells "global hypomethylation" due to disruption in DNA methyltransferases (DNMTs) may promote mitotic recombination and chromosome rearrangement, ultimately resulting in aneuploidy when the chromosomes fail to separate properly during mitosis.[14][15][16][17]
CpG island methylation is important in regulation of gene expression, yet cytosine methylation can lead directly to destabilizing genetic mutations and a precancerous cellular state. Methylated cytosines make hydrolysis of the amine group and spontaneous conversion to thymine more favorable. They can cause aberrant recruitment of chromatin proteins. Cytosine methylations change the amount of UV light absorption of the nucleotide base, creating pyrimidine dimers. When mutation results in loss of heterozygosity at tumor suppressor gene sites, these genes may become inactive. Single base pair mutations during replication can also have detrimental effects.[12]
Histone modification
Eukaryotic DNA has a complex structure. It is generally wrapped around special proteins called histones to form a structure called a nucleosome. A nucleosome consists of 2 sets of 4 histones: H2A, H2B, H3, and H4. Additionally, histone H1 contributes to DNA packaging outside of the nucleosome. Certain histone modifying enzymes can add or remove functional groups to the histones, and these modifications influence the level of transcription of the genes wrapped around those histones and the level of DNA replication. Histone modification profiles of healthy and cancerous cells tend to differ.
In comparison to healthy cells, cancerous cells exhibit decreased monoacetylated and trimethylated forms of histone H4 (decreased H4ac and H4me3).[22] Additionally, mouse models have shown that a decrease in histone H4R3 asymmetric dimethylation (H4R3me2a) of the p19ARF promoter is correlated with more advanced cases of tumorigenesis and metastasis.[23] In mouse models, the loss of histone H4 acetylation and trimethylation increases as tumor growth continues.[22] Loss of histone H4 Lysine 16 acetylation (H4K16ac), which is a mark of aging at the telomeres, specifically loses its acetylation. Some scientists hope this particular loss of histone acetylation might be battled with a histone deacetylase (HDAC) inhibitor specific for SIRT1, an HDAC specific for H4K16.[10][24]
Other histone marks associated with tumorigenesis include increased deacetylation (decreased acetylation) of histones H3 and H4, decreased trimethylation of histone H3 Lysine 4 (H3K4me3), and increased monomethylation of histone H3 Lysine 9 (H3K9me1) and trimethylation of histone H3 Lysine 27 (H3K27me3). These histone modifications can silence tumor suppressor genes despite the drop in methylation of the gene's CpG island (an event that normally activates genes).[25][26]
Some research has focused on blocking the action of BRD4 on acetylated histones, which has been shown to increase the expression of the Myc protein, implicated in several cancers. The development process of the drug to bind to BRD4 is noteworthy for the collaborative, open approach the team is taking.[27]
The tumor suppressor gene p53 regulates DNA repair and can induce apoptosis in dysregulated cells. E Soto-Reyes and F Recillas-Targa elucidated the importance of the CTCF protein in regulating p53 expression.[28] CTCF, or CCCTC binding factor, is a zinc finger protein that insulates the p53 promoter from accumulating repressive histone marks. In certain types of cancer cells, the CTCF protein does not bind normally, and the p53 promoter accumulates repressive histone marks, causing p53 expression to decrease.[28]
Mutations in the epigenetic machinery itself may occur as well, potentially responsible for the changing epigenetic profiles of cancerous cells. The histone variants of the H2A family are highly conserved in mammals, playing critical roles in regulating many nuclear processes by altering chromatin structure. One of the key H2A variants, H2A.X, marks DNA damage, facilitating the recruitment of DNA repair proteins to restore genomic integrity. Another variant, H2A.Z, plays an important role in both gene activation and repression. A high level of H2A.Z expression is detected in many cancers and is significantly associated with cellular proliferation and genomic instability.[11] Histone variant macroH2A1 is important in the pathogenesis of many types of cancers, for instance in hepatocellular carcinoma.[29] Other mechanisms include a decrease in H4K16ac may be caused by either a decrease in activity of a histone acetyltransferases (HATs) or an increase in deacetylation by SIRT1.[10] Likewise, an inactivating frameshift mutation in HDAC2, a histone deacetylase that acts on many histone-tail lysines, has been associated with cancers showing altered histone acetylation patterns.[30] These findings indicate a promising mechanism for altering epigenetic profiles through enzymatic inhibition or enhancement. A new emerging field that captures toxicological epigenetic changes as a result of the exposure to different compounds (drugs, food, and environment) is toxicoepigenetics. In this field, there is growing interest in mapping changes in histone modifications and their possible consequences.[31]
DNA damage, caused by UV light, ionizing radiation, environmental toxins, and metabolic chemicals, can also lead to genomic instability and cancer. The DNA damage response to double strand DNA breaks (DSB) is mediated in part by histone modifications. At a DSB, MRE11-RAD50-NBS1 (MRN) protein complex recruits ataxia telangiectasia mutated (ATM) kinase which phosphorylates Serine 129 of Histone 2A. MDC1, mediator of DNA damage checkpoint 1, binds to the phosphopeptide, and phosphorylation of H2AX may spread by a positive feedback loop of MRN-ATM recruitment and phosphorylation. TIP60 acetylates the γH2AX, which is then polyubiquitylated. RAP80, a subunit of the DNA repair breast cancer type 1 susceptibility protein complex (BRCA1-A), binds ubiquitin attached to histones. BRCA1-A activity arrests the cell cycle at the G2/M checkpoint, allowing time for DNA repair, or apoptosis may be initiated.[32]
MicroRNA gene silencing
In mammals, microRNAs (miRNAs) regulate about 60% of the transcriptional activity of protein-encoding genes.[33] Some miRNAs also undergo methylation-associated silencing in cancer cells.[34][35] Let-7 and miR15/16 play important roles in down-regulating RAS and BCL2 oncogenes, and their silencing occurs in cancer cells.[18] Decreased expression of miR-125b1, a miRNA that functions as a tumor suppressor, was observed in prostate, ovarian, breast and glial cell cancers. In vitro experiments have shown that miR-125b1 targets two genes, HER2/neu and ESR1, that are linked to breast cancer. DNA methylation, specifically hypermethylation, is one of the main ways that the miR-125b1 is epigenetically silenced. In patients with breast cancer, hypermethylation of CpG islands located proximal to the transcription start site was observed. Loss of CTCF binding and an increase in repressive histone marks, H3K9me3 and H3K27me3, correlates with DNA methylation and miR-125b1 silencing. Mechanistically, CTCF may function as a boundary element to stop the spread of DNA methylation. Results from experiments conducted by Soto-Reyes et al.[36] indicate a negative effect of methylation on the function and expression of miR-125b1. Therefore, they concluded that DNA methylation has a part in silencing the gene. Furthermore, some miRNA's are epigenetically silenced early on in breast cancer, and therefore these miRNA's could potentially be useful as tumor markers.[36] The epigenetic silencing of miRNA genes by aberrant DNA methylation is a frequent event in cancer cells; almost one third of miRNA promoters active in normal mammary cells were found hypermethylated in breast cancer cells - that is a several fold greater proportion than is usually observed for protein coding genes.[37]
Metabolic recoding of epigenetics in cancer
Dysregulation of metabolism allows tumor cells to generate needed building blocks as well as to modulate epigenetic marks to support cancer initiation and progression. Cancer-induced metabolic changes alter the epigenetic landscape, especially modifications on histones and DNA, thereby promoting malignant transformation, adaptation to inadequate nutrition, and metastasis. In order to satisfy the biosynthetic demands of cancer cells, metabolic pathways are altered by manipulating oncogenes and tumor suppressive genes concurrently.[38] The accumulation of certain metabolites in cancer can target epigenetic enzymes to globally alter the epigenetic landscape. Cancer-related metabolic changes lead to locus-specific recoding of epigenetic marks. Cancer epigenetics can be precisely reprogramed by cellular metabolism through 1) dose-responsive modulation of cancer epigenetics by metabolites; 2) sequence-specific recruitment of metabolic enzymes; and 3) targeting of epigenetic enzymes by nutritional signals.[38] In addition to modulating metabolic programming on a molecular level, there are microenvironmental factors that can influence and effect metabolic recoding. These influences include nutritional, inflammatory, and the immune response of malignant tissues.
MicroRNA and DNA repair
DNA damage appears to be the primary underlying cause of cancer.[39][40] If DNA repair is deficient, DNA damage tends to accumulate. Such excess DNA damage can increase mutational errors during DNA replication due to error-prone translesion synthesis. Excess DNA damage can also increase epigenetic alterations due to errors during DNA repair.[41][42] Such mutations and epigenetic alterations can give rise to cancer (see malignant neoplasms).
Germ line mutations in DNA repair genes cause only 2–5% of colon cancer cases.[43] However, altered expression of microRNAs, causing DNA repair deficiencies, are frequently associated with cancers and may be an important causal factor for these cancers.
Over-expression of certain miRNAs may directly reduce expression of specific DNA repair proteins. Wan et al.[44] referred to 6 DNA repair genes that are directly targeted by the miRNAs indicated in parentheses: ATM (miR-421), RAD52 (miR-210, miR-373), RAD23B (miR-373), MSH2 (miR-21), BRCA1 (miR-182) and P53 (miR-504, miR-125b). More recently, Tessitore et al.[45] listed further DNA repair genes that are directly targeted by additional miRNAs, including ATM (miR-18a, miR-101), DNA-PK (miR-101), ATR (miR-185), Wip1 (miR-16), MLH1, MSH2 and MSH6 (miR-155), ERCC3 and ERCC4 (miR-192) and UNG2 (mir-16, miR-34c and miR-199a). Of these miRNAs, miR-16, miR-18a, miR-21, miR-34c, miR-125b, miR-101, miR-155, miR-182, miR-185 and miR-192 are among those identified by Schnekenburger and Diederich[46] as over-expressed in colon cancer through epigenetic hypomethylation. Over expression of any one of these miRNAs can cause reduced expression of its target DNA repair gene.
Up to 15% of the MLH1-deficiencies in sporadic colon cancers appeared to be due to over-expression of the microRNA miR-155, which represses MLH1 expression.[47] However, the majority of 68 sporadic colon cancers with reduced expression of the DNA mismatch repair protein MLH1 were found to be deficient due to epigenetic methylation of the CpG island of the MLH1 gene.[48]
In 28% of glioblastomas, the MGMT DNA repair protein is deficient but the MGMT promoter is not methylated.[49] In the glioblastomas without methylated MGMT promoters, the level of microRNA miR-181d is inversely correlated with protein expression of MGMT and the direct target of miR-181d is the MGMT mRNA 3'UTR (the three prime untranslated region of MGMT mRNA).[49] Thus, in 28% of glioblastomas, increased expression of miR-181d and reduced expression of DNA repair enzyme MGMT may be a causal factor. In 29–66%[49][50] of glioblastomas, DNA repair is deficient due to epigenetic methylation of the MGMT gene, which reduces protein expression of MGMT.
High mobility group A (HMGA) proteins, characterized by an AT-hook, are small, nonhistone, chromatin-associated proteins that can modulate transcription. MicroRNAs control the expression of HMGA proteins, and these proteins (HMGA1 and HMGA2) are architectural chromatin transcription-controlling elements. Palmieri et al.[51] showed that, in normal tissues, HGMA1 and HMGA2 genes are targeted (and thus strongly reduced in expression) by miR-15, miR-16, miR-26a, miR-196a2 and Let-7a.
HMGA expression is almost undetectable in differentiated adult tissues but is elevated in many cancers. HGMA proteins are polypeptides of ~100 amino acid residues characterized by a modular sequence organization. These proteins have three highly positively charged regions, termed AT hooks, that bind the minor groove of AT-rich DNA stretches in specific regions of DNA. Human neoplasias, including thyroid, prostatic, cervical, colorectal, pancreatic and ovarian carcinoma, show a strong increase of HMGA1a and HMGA1b proteins.[52] Transgenic mice with HMGA1 targeted to lymphoid cells develop aggressive lymphoma, showing that high HMGA1 expression is not only associated with cancers, but that the HMGA1 gene can act as an oncogene to cause cancer.[53] Baldassarre et al.,[54] showed that HMGA1 protein binds to the promoter region of DNA repair gene BRCA1 and inhibits BRCA1 promoter activity. They also showed that while only 11% of breast tumors had hypermethylation of the BRCA1 gene, 82% of aggressive breast cancers have low BRCA1 protein expression, and most of these reductions were due to chromatin remodeling by high levels of HMGA1 protein.
HMGA2 protein specifically targets the promoter of ERCC1, thus reducing expression of this DNA repair gene.[55] ERCC1 protein expression was deficient in 100% of 47 evaluated colon cancers (though the extent to which HGMA2 was involved is unknown).[56]
Palmieri et al.[51] showed that each of the miRNAs that target HMGA genes are drastically reduced in almost all human pituitary adenomas studied, when compared with the normal pituitary gland. Consistent with the down-regulation of these HMGA-targeting miRNAs, an increase in the HMGA1 and HMGA2-specific mRNAs was observed. Three of these microRNAs (miR-16, miR-196a and Let-7a)[46][57] have methylated promoters and therefore low expression in colon cancer. For two of these, miR-15 and miR-16, the coding regions are epigenetically silenced in cancer due to histone deacetylase activity.[58] When these microRNAs are expressed at a low level, then HMGA1 and HMGA2 proteins are expressed at a high level. HMGA1 and HMGA2 target (reduce expression of) BRCA1 and ERCC1 DNA repair genes. Thus DNA repair can be reduced, likely contributing to cancer progression.[40]
DNA repair pathways

The chart in this section shows some frequent DNA damaging agents, examples of DNA lesions they cause, and the pathways that deal with these DNA damages. At least 169 enzymes are either directly employed in DNA repair or influence DNA repair processes.[59] Of these, 83 are directly employed in repairing the 5 types of DNA damages illustrated in the chart.
Some of the more well studied genes central to these repair processes are shown in the chart. The gene designations shown in red, gray or cyan indicate genes frequently epigenetically altered in various types of cancers. Wikipedia articles on each of the genes highlighted by red, gray or cyan describe the epigenetic alteration(s) and the cancer(s) in which these epimutations are found. Two broad experimental survey articles[60][61] also document most of these epigenetic DNA repair deficiencies in cancers.
Red-highlighted genes are frequently reduced or silenced by epigenetic mechanisms in various cancers. When these genes have low or absent expression, DNA damages can accumulate. Replication errors past these damages (see translesion synthesis) can lead to increased mutations and, ultimately, cancer. Epigenetic repression of DNA repair genes in accurate DNA repair pathways appear to be central to carcinogenesis.
The two gray-highlighted genes RAD51 and BRCA2, are required for homologous recombinational repair. They are sometimes epigenetically over-expressed and sometimes under-expressed in certain cancers. As indicated in the Wikipedia articles on RAD51 and BRCA2, such cancers ordinarily have epigenetic deficiencies in other DNA repair genes. These repair deficiencies would likely cause increased unrepaired DNA damages. The over-expression of RAD51 and BRCA2 seen in these cancers may reflect selective pressures for compensatory RAD51 or BRCA2 over-expression and increased homologous recombinational repair to at least partially deal with such excess DNA damages. In those cases where RAD51 or BRCA2 are under-expressed, this would itself lead to increased unrepaired DNA damages. Replication errors past these damages (see translesion synthesis) could cause increased mutations and cancer, so that under-expression of RAD51 or BRCA2 would be carcinogenic in itself.
Cyan-highlighted genes are in the microhomology-mediated end joining (MMEJ) pathway and are up-regulated in cancer. MMEJ is an additional error-prone inaccurate repair pathway for double-strand breaks. In MMEJ repair of a double-strand break, an homology of 5-25 complementary base pairs between both paired strands is sufficient to align the strands, but mismatched ends (flaps) are usually present. MMEJ removes the extra nucleotides (flaps) where strands are joined, and then ligates the strands to create an intact DNA double helix. MMEJ almost always involves at least a small deletion, so that it is a mutagenic pathway.[62] FEN1, the flap endonuclease in MMEJ, is epigenetically increased by promoter hypomethylation and is over-expressed in the majority of cancers of the breast,[63] prostate,[64] stomach,[65][66] neuroblastomas,[67] pancreas,[68] and lung.[69] PARP1 is also over-expressed when its promoter region ETS site is epigenetically hypomethylated, and this contributes to progression to endometrial cancer,[70] BRCA-mutated ovarian cancer,[71] and BRCA-mutated serous ovarian cancer.[72] Other genes in the MMEJ pathway are also over-expressed in a number of cancers (see MMEJ for summary), and are also shown in blue.
Frequencies of epimutations in DNA repair genes
Deficiencies in DNA repair proteins that function in accurate DNA repair pathways increase the risk of mutation. Mutation rates are strongly increased in cells with mutations in DNA mismatch repair[73][74] or in homologous recombinational repair (HRR).[75] Individuals with inherited mutations in any of 34 DNA repair genes are at increased risk of cancer (see DNA repair defects and increased cancer risk).
In sporadic cancers, a deficiency in DNA repair is occasionally found to be due to a mutation in a DNA repair gene, but much more frequently reduced or absent expression of DNA repair genes is due to epigenetic alterations that reduce or silence gene expression. For example, for 113 colorectal cancers examined in sequence, only four had a missense mutation in the DNA repair gene MGMT, while the majority had reduced MGMT expression due to methylation of the MGMT promoter region (an epigenetic alteration).[76] Similarly, out of 119 cases of mismatch repair-deficient colorectal cancers that lacked DNA repair gene PMS2 expression, PMS2 protein was deficient in 6 due to mutations in the PMS2 gene, while in 103 cases PMS2 expression was deficient because its pairing partner MLH1 was repressed due to promoter methylation (PMS2 protein is unstable in the absence of MLH1).[48] In the other 10 cases, loss of PMS2 expression was likely due to epigenetic overexpression of the microRNA, miR-155, which down-regulates MLH1.[47]
Epigenetic defects in DNA repair genes are frequent in cancers. In the table, multiple cancers were evaluated for reduced or absent expression of the DNA repair gene of interest, and the frequency shown is the frequency with which the cancers had an epigenetic deficiency of gene expression. Such epigenetic deficiencies likely arise early in carcinogenesis, since they are also frequently found (though at somewhat lower frequency) in the field defect surrounding the cancer from which the cancer likely arose (see Table).
Cancer | Gene | Frequency in Cancer | Frequency in Field Defect | Ref. |
---|---|---|---|---|
Colorectal | MGMT | 46% | 34% | [77] |
Colorectal | MGMT | 47% | 11% | [78] |
Colorectal | MGMT | 70% | 60% | [79] |
Colorectal | MSH2 | 13% | 5% | [78] |
Colorectal | ERCC1 | 100% | 40% | [56] |
Colorectal | PMS2 | 88% | 50% | [56] |
Colorectal | XPF | 55% | 40% | [56] |
Head and Neck | MGMT | 54% | 38% | [80] |
Head and Neck | MLH1 | 33% | 25% | [81] |
Head and Neck | MLH1 | 31% | 20% | [82] |
Stomach | MGMT | 88% | 78% | [83] |
Stomach | MLH1 | 73% | 20% | [84] |
Stomach | ERCC1 | 95-100% | 14-65% | [85] |
Stomach | PMS2 | 95-100% | 14-60% | [85] |
Esophagus | MLH1 | 77%–100% | 23%–79% | [86] |
It appears that cancers may frequently be initiated by an epigenetic reduction in expression of one or more DNA repair enzymes. Reduced DNA repair likely allows accumulation of DNA damages. Error prone translesion synthesis past some of these DNA damages may give rise to a mutation with a selective advantage. A clonal patch with a selective advantage may grow and out-compete neighboring cells, forming a field defect. While there is no obvious selective advantage for a cell to have reduced DNA repair, the epimutation of the DNA repair gene may be carried along as a passenger when the cells with the selectively advantageous mutation are replicated. In the cells carrying both the epimutation of the DNA repair gene and the mutation with the selective advantage, further DNA damages will accumulate, and these could, in turn, give rise to further mutations with still greater selective advantages. Epigenetic defects in DNA repair may thus contribute to the characteristic high frequency of mutations in the genomes of cancers, and cause their carcinogenic progression.
Cancers have high levels of genome instability, associated with a high frequency of mutations. A high frequency of genomic mutations increases the likelihood of particular mutations occurring that activate oncogenes and inactivate tumor suppressor genes, leading to carcinogenesis. On the basis of whole genome sequencing, cancers are found to have thousands to hundreds of thousands of mutations in their whole genomes.[87] (Also see Mutation frequencies in cancers.) By comparison, the mutation frequency in the whole genome between generations for humans (parent to child) is about 70 new mutations per generation.[88][89] In the protein coding regions of the genome, there are only about 0.35 mutations between parent/child generations (less than one mutated protein per generation).[90] Whole genome sequencing in blood cells for a pair of identical twin 100-year-old centenarians only found 8 somatic differences, though somatic variation occurring in less than 20% of blood cells would be undetected.[91]
While DNA damages may give rise to mutations through error prone translesion synthesis, DNA damages can also give rise to epigenetic alterations during faulty DNA repair processes.[41][42][92][93] The DNA damages that accumulate due to epigenetic DNA repair defects can be a source of the increased epigenetic alterations found in many genes in cancers. In an early study, looking at a limited set of transcriptional promoters, Fernandez et al.[94] examined the DNA methylation profiles of 855 primary tumors. Comparing each tumor type with its corresponding normal tissue, 729 CpG island sites (55% of the 1322 CpG sites evaluated) showed differential DNA methylation. Of these sites, 496 were hypermethylated (repressed) and 233 were hypomethylated (activated). Thus, there is a high level of epigenetic promoter methylation alterations in tumors. Some of these epigenetic alterations may contribute to cancer progression.
Epigenetic carcinogens
A variety of compounds are considered as epigenetic carcinogens—they result in an increased incidence of tumors, but they do not show mutagen activity (toxic compounds or pathogens that cause tumors incident to increased regeneration should also be excluded). Examples include diethylstilbestrol, arsenite, hexachlorobenzene, and nickel compounds.
Many teratogens exert specific effects on the fetus by epigenetic mechanisms.[95][96] While epigenetic effects may preserve the effect of a teratogen such as diethylstilbestrol throughout the life of an affected child, the possibility of birth defects resulting from exposure of fathers or in second and succeeding generations of offspring has generally been rejected on theoretical grounds and for lack of evidence.[97] However, a range of male-mediated abnormalities have been demonstrated, and more are likely to exist.[98] FDA label information for Vidaza, a formulation of 5-azacitidine (an unmethylatable analog of cytidine that causes hypomethylation when incorporated into DNA) states that "men should be advised not to father a child" while using the drug, citing evidence in treated male mice of reduced fertility, increased embryo loss, and abnormal embryo development.[99] In rats, endocrine differences were observed in offspring of males exposed to morphine.[100] In mice, second generation effects of diethylstilbesterol have been described occurring by epigenetic mechanisms.[101]
Cancer subtypes
Skin cancer
Melanoma is a deadly skin cancer that originates from melanocytes. Several epigenetic alterations are known to play a role in the transition of melanocytes to melanoma cells. This includes DNA methylation that can be inherited without making changes to the DNA sequence, as well as silencing the tumor suppressor genes in the epidermis that have been exposed to UV radiation for periods of time.[102] The silencing of tumor suppressor genes leads to photocarcinogenesis which is associated to epigenetic alterations in DNA methylation, DNA methyltransferases, and histone acetylation.[102] These alterations are the consequence of deregulation of their corresponding enzymes. Several histone methyltransferases and demethylases are among these enzymes.[103]
Prostate cancer
Prostate cancer kills around 35,000 men yearly, and about 220,000 men are diagnosed with prostate cancer per year, in North America alone.[104] Prostate cancer is the second leading cause of cancer-caused fatalities in men, and within a man's lifetime, one in six men will have the disease.[104] Alterations in histone acetylation and DNA methylation occur in various genes influencing prostate cancer, and have been seen in genes involved in hormonal response.[105] More than 90% of prostate cancers show gene silencing by CpG island hypermethylation of the GSTP1 gene promoter, which protects prostate cells from genomic damage that is caused by different oxidants or carcinogens.[106] Real-time methylation-specific polymerase chain reaction (PCR) suggests that many other genes are also hypermethylated.[106] Gene expression in the prostate may be modulated by nutrition and lifestyle changes.[107]
Cervical cancer
The second most common malignant tumor in women is invasive cervical cancer (ICC) and more than 50% of all invasive cervical cancer (ICC) is caused by oncongenic human papillomavirus 16 (HPV16).[108] Furthermore, cervix intraepithelial neoplasia (CIN) is primarily caused by oncogenic HPV16.[108] As in many cases, the causative factor for cancer does not always take a direct route from infection to the development of cancer. Genomic methylation patterns have been associated with invasive cervical cancer. Within the HPV16L1 region, 14 tested CpG sites have significantly higher methylation in CIN3+ than in HPV16 genomes of women without CIN3.[108] Only 2/16 CpG sites tested in HPV16 upstream regulatory region were found to have association with increased methylation in CIN3+.[108] This suggests that the direct route from infection to cancer is sometimes detoured to a precancerous state in cervix intraepithelial neoplasia. Additionally, increased CpG site methylation was found in low levels in most of the five host nuclear genes studied, including 5/5 TERT, 1/4 DAPK1, 2/5 RARB, MAL, and CADM1.[108] Furthermore, 1/3 of CpG sites in mitochondrial DNA were associated with increased methylation in CIN3+.[108] Thus, a correlation exists between CIN3+ and increased methylation of CpG sites in the HPV16 L1 open reading frame.[108] This could be a potential biomarker for future screens of cancerous and precancerous cervical disease.[108]
Leukemia
Recent studies have shown that the mixed-lineage leukemia (MLL) gene causes leukemia by rearranging and fusing with other genes in different chromosomes, which is a process under epigenetic control.[109] Mutations in MLL block the correct regulatory regions in leukemia associated translocations or insertions causing malignant transformation controlled by HOX genes.[110] This is what leads to the increase in white blood cells. Leukemia related genes are managed by the same pathways that control epigenetics, signaling transduction, transcriptional regulation, and energy metabolism. It was indicated that infections, electromagnetic fields and increased birth weight can contribute to being the causes of leukemia.[111]
Sarcoma
There are about 15,000 new cases of sarcoma in the US each year, and about 6,200 people were projected to die of sarcoma in the US in 2014.[112] Sarcomas comprise a large number of rare, histogenetically heterogeneous mesenchymal tumors that, for example, include chondrosarcoma, Ewing's sarcoma, leiomyosarcoma, liposarcoma, osteosarcoma, synovial sarcoma, and (alveolar and embryonal) rhabdomyosarcoma. Several oncogenes and tumor suppressor genes are epigenetically altered in sarcomas. These include APC, CDKN1A, CDKN2A, CDKN2B, Ezrin, FGFR1, GADD45A, MGMT, STK3, STK4, PTEN, RASSF1A, WIF1, as well as several miRNAs.[113] Expression of epigenetic modifiers such as that of the BMI1 component of the PRC1 complex is deregulated in chondrosarcoma, Ewing's sarcoma, and osteosarcoma, and expression of the EZH2 component of the PRC2 complex is altered in Ewing's sarcoma and rhabdomyosarcoma. Similarly, expression of another epigenetic modifier, the LSD1 histone demethylase, is increased in chondrosarcoma, Ewing's sarcoma, osteosarcoma, and rhabdomyosarcoma. Drug targeting and inhibition of EZH2 in Ewing's sarcoma,[114] or of LSD1 in several sarcomas,[115] inhibits tumor cell growth in these sarcomas.
Lung Cancer
Lung cancer is the second most common type of cancer and leading cause of death in men and women in the United States, it is estimated that there is about 216,000 new cases and 160,000 deaths due to lung cancer.[116]
Initiation and progression of lung carcinoma is the result of the interaction between genetic, epigenetic and environmental factors. Most cases of lung cancer are because of genetic mutations in EGFR, KRAS, STK11 (also known as LKB1), TP53 (also known as p53), and CDKN2A (also known as p16 or INK4a)[117][118][119] with the most common type of lung cancer being an inactivation at p16. p16 is a tumor suppressor protein that occurs in mostly in humans the functional significance of the mutations was tested on many other species including mice, cats, dogs, monkeys and cows the identification of these multiple nonoverlapping clones was not entirely surprising since the reduced stringency hybridization of a zoo blot with the same probe also revealed 10-15 positive EcoRI fragments in all species tested.[120]
Identification methods
Previously, epigenetic profiles were limited to individual genes under scrutiny by a particular research team. Recently, however, scientists have been moving toward a more genomic approach to determine an entire genomic profile for cancerous versus healthy cells.[10]
Popular approaches for measuring CpG methylation in cells include:
- Bisulfite sequencing
- Combined bisulfite restriction analysis (COBRA)
- Methylation-specific PCR
- MethyLight
- Pyrosequencing
- Restriction landmark genomic scanning
- Arbitrary primed PCR
- HELP assay (HpaII tiny fragment enrichment by ligation-mediated PCR)
- Chromatin immunoprecipitation ChIP-Chip using antibodies specific for methyl-CpG binding domain proteins
- Methylated DNA immunoprecipitation Methyl-DIP
- Gene-expression profiles via DNA microarray : comparing mRNA levels from cancer cell lines before and after treatment with a demethylating agent
Since bisulfite sequencing is considered the gold standard for measuring CpG methylation, when one of the other methods is used, results are usually confirmed using bisulfite sequencing[1]. Popular approaches for determining histone modification profiles in cancerous versus healthy cells include:[10]
- Mass spectrometry
- Chromatin Immunoprecipitation Assay
Diagnosis and prognosis
Researchers are hoping to identify specific epigenetic profiles of various types and subtypes of cancer with the goal of using these profiles as tools to diagnose individuals more specifically and accurately.[10] Since epigenetic profiles change, scientists would like to use the different epigenomic profiles to determine the stage of development or level of aggressiveness of a particular cancer in patients. For example, hypermethylation of the genes coding for Death-Associated Protein Kinase (DAPK), p16, and Epithelial Membrane Protein 3 (EMP3) have been linked to more aggressive forms of lung, colorectal, and brain cancers.[17] This type of knowledge can affect the way that doctors will diagnose and choose to treat their patients.
Another factor that will influence the treatment of patients is knowing how well they will respond to certain treatments. Personalized epigenomic profiles of cancerous cells can provide insight into this field. For example, MGMT is an enzyme that reverses the addition of alkyl groups to the nucleotide guanine.[121] Alkylating guanine, however, is the mechanism by which several chemotherapeutic drugs act in order to disrupt DNA and cause cell death.[122][123][124][125] Therefore, if the gene encoding MGMT in cancer cells is hypermethylated and in effect silenced or repressed, the chemotherapeutic drugs that act by methylating guanine will be more effective than in cancer cells that have a functional MGMT enzyme.
Epigenetic biomarkers can also be utilized as tools for molecular prognosis. In primary tumor and mediastinal lymph node biopsy samples, hypermethylation of both CDKN2A and CDH13 serves as the marker for increased risk of faster cancer relapse and higher death rate of patients.[126]
Treatment
Epigenetic control of the proto-onco regions and the tumor suppressor sequences by conformational changes in histones plays a role in the formation and progression of cancer.[127] Pharmaceuticals that reverse epigenetic changes might have a role in a variety of cancers.[105][127][128]
Recently, it is evidently known that associations between specific cancer histotypes and epigenetic changes can facilitate the development of novel epi-drugs.[129] Drug development has focused mainly on modifying DNA methyltransferase, histone acetyltransferase (HAT) and histone deacetylase (HDAC).[130]
Drugs that specifically target the inverted methylation pattern of cancerous cells include the DNA methyltransferase inhibitors azacitidine[131][132] and decitabine.[133][134] These hypomethylating agents are used to treat myelodysplastic syndrome,[135] a blood cancer produced by abnormal bone marrow stem cells.[12] These agents inhibit all three types of active DNA methyltransferases, and had been thought to be highly toxic, but proved to be effective when used in low dosage, reducing progression of myelodysplastic syndrome to leukemia.[136]
Histone deacetylase (HDAC) inhibitors show efficacy in treatment of T cell lymphoma. two HDAC inhibitors, vorinostat and romidepsin, have been approved by the Food and Drug Administration.[137][138] However, since these HDAC inhibitors alter the acetylation state of many proteins in addition to the histone of interest, knowledge of the underlying mechanism at the molecular level of patient response is required to enhance the efficiency of using such inhibitors as treatment.[18] Treatment with HDAC inhibitors has been found to promote gene reactivation after DNA methyl-transferases inhibitors have repressed transcription.[139] Panobinostat is approved for certain situations in myeloma.[140]
Other pharmaceutical targets in research are histone lysine methyltransferases (KMT) and protein arginine methyltransferases (PRMT).[141] Preclinical study has suggested that lunasin may have potentially beneficial epigenetic effects.[142]
Epigenetic Therapy
Epigenetic therapy of cancer has shown to be a promising and possible treatment of cancerous cells. Epigenetic inactivation is an ideal target for cancerous cells because it targets genes imperative for controlling cell growth, specifically cancer cell growth. It is crucial for these genes to be reactivated in order to suppress tumor growth and sensitize the cells to cancer curing therapies.[143] Typical chemotherapy aims to kill and eliminate cancer cells in the body. Cancer initiated by genetic alterations of cells are typically permanent and nearly impossible to reverse, this differs from epigenetic cancer because the cancer causing epigenetic aberrations have the capability of being reversed, and the cells being returned to normal function. The ability for epigenetic mechanisms to be reversed is attributed to the fact that the coding of the genes being silenced through histone and DNA modification is not being altered.[144]
There are two primary types of epigenetic alterations in cancer cells, these are known as DNA methylation and Histone modification. It is the goal of epigenetic therapies to inhibit these alterations. DNA Methyltransferases (DNMTs) and Histone Deacetylases (HDAC) are the primary catalyzes of the epigenetic modifications of cancer cells.[145] The goal for epigenetic therapies is to repress this methylation and reverse these modifications in order to create a new epigenome where cancer cells no longer thrive and tumor suppression is the new function. Synthetic drugs are used as tools in epigenetic therapies due to their ability to inhibit enzymes causing histone modifications and DNA methylations. Combination therapy is one method of epigenetic therapy which involves the use of more than one synthetic drug, these drugs include a low dose DNMT inhibitor as well as an HDAC inhibitor. Together, these drugs are able to target the linkage between DNA methylation and Histone modification.[146]
The goal of epigenetic therapies for cancer in relation to DNA methylation is to both decrease the methylation of DNA and in turn decrease the silencing of genes related to tumor suppression.[147] The term associated with decreasing the methylation of DNA will be known as hypomethylation. The Food and Drug Administration (FDA) has currently approved one hypomethylating agent which, through the conduction of clinical trials, has shown promising results when utilized to treat patients with Myelodysplastic Syndrome (MDS).[148] This hypomethylating agent is known as the doozy analogue of 5-azacytidine and works to promote hypomethylation by targeting all DNA methyltransferases for degradation.[147]
See also
References
- ↑ "Epigenetics in cancer". Carcinogenesis 31 (1): 27–36. January 2010. doi:10.1093/carcin/bgp220. PMID 19752007.
- ↑ "Cancer genome landscapes". Science 339 (6127): 1546–1558. March 2013. doi:10.1126/science.1235122. PMID 23539594. Bibcode: 2013Sci...339.1546V.
- ↑ "Orphan CpG islands identify numerous conserved promoters in the mammalian genome". PLOS Genetics 6 (9): e1001134. September 2010. doi:10.1371/journal.pgen.1001134. PMID 20885785.
- ↑ "Discovery and Validation of Hypermethylated Markers for Colorectal Cancer". Disease Markers 2016: 2192853. 2016. doi:10.1155/2016/2192853. PMID 27493446.
- ↑ "Whole-genome methylation analysis of benign and malignant colorectal tumours". The Journal of Pathology 229 (5): 697–704. April 2013. doi:10.1002/path.4132. PMID 23096130.
- ↑ "Epigenetics changes in cancer cells". MedGenMed 6 (4): 17. December 2004. PMID 15775844.
- ↑ "Epimutation and cancer: a new carcinogenic mechanism of Lynch syndrome (Review)". International Journal of Oncology 41 (3): 793–797. September 2012. doi:10.3892/ijo.2012.1528. PMID 22735547.
- ↑ "DNA methylation patterns and epigenetic memory". Genes & Development 16 (1): 6–21. January 2002. doi:10.1101/gad.947102. PMID 11782440.
- ↑ "Methylation-specific PCR: a novel PCR assay for methylation status of CpG islands". Proceedings of the National Academy of Sciences of the United States of America 93 (18): 9821–9826. September 1996. doi:10.1073/pnas.93.18.9821. PMID 8790415. Bibcode: 1996PNAS...93.9821H.
- ↑ 10.0 10.1 10.2 10.3 10.4 10.5 10.6 10.7 "Cancer epigenomics: DNA methylomes and histone-modification maps". Nature Reviews. Genetics 8 (4): 286–298. April 2007. doi:10.1038/nrg2005. PMID 17339880.
- ↑ 11.0 11.1 Epigenetics: A Reference Manual. Norfolk, England: Caister Academic Press. 2011. ISBN 978-1-904455-88-2.
- ↑ 12.0 12.1 12.2 "The fundamental role of epigenetic events in cancer". Nature Reviews. Genetics 3 (6): 415–428. June 2002. doi:10.1038/nrg816. PMID 12042769.
- ↑ "DNA methylation screening identifies driver epigenetic events of cancer cell survival". Cancer Cell 21 (5): 655–667. May 2012. doi:10.1016/j.ccr.2012.03.045. PMID 22624715.
- ↑ 14.0 14.1 14.2 "Gene silencing in cancer in association with promoter hypermethylation". The New England Journal of Medicine 349 (21): 2042–2054. November 2003. doi:10.1056/NEJMra023075. PMID 14627790.
- ↑ 15.0 15.1 15.2 "The history of cancer epigenetics". Nature Reviews. Cancer 4 (2): 143–153. February 2004. doi:10.1038/nrc1279. PMID 14732866.
- ↑ 16.0 16.1 16.2 "Epigenetics in human disease and prospects for epigenetic therapy". Nature 429 (6990): 457–463. May 2004. doi:10.1038/nature02625. PMID 15164071. Bibcode: 2004Natur.429..457E.
- ↑ 17.0 17.1 17.2 17.3 "Aberrant DNA methylation as a cancer-inducing mechanism". Annual Review of Pharmacology and Toxicology 45: 629–656. 2005. doi:10.1146/annurev.pharmtox.45.120403.095832. PMID 15822191.
- ↑ 18.0 18.1 18.2 "A decade of exploring the cancer epigenome - biological and translational implications". Nature Reviews. Cancer 11 (10): 726–734. September 2011. doi:10.1038/nrc3130. PMID 21941284.
- ↑ "RNAi and heterochromatin repress centromeric meiotic recombination". Proceedings of the National Academy of Sciences of the United States of America 107 (19): 8701–8705. May 2010. doi:10.1073/pnas.0914160107. PMID 20421495. Bibcode: 2010PNAS..107.8701E.
- ↑ "Cancer epigenomics: DNA methylomes and histone-modification maps" (in En). Nature Reviews. Genetics 8 (4): 286–298. April 2007. doi:10.1038/nrg2005. PMID 17339880.
- ↑ "Cancer as a dysregulated epigenome allowing cellular growth advantage at the expense of the host" (in En). Nature Reviews. Cancer 13 (7): 497–510. July 2013. doi:10.1038/nrc3486. PMID 23760024.
- ↑ 22.0 22.1 "Loss of acetylation at Lys16 and trimethylation at Lys20 of histone H4 is a common hallmark of human cancer". Nature Genetics 37 (4): 391–400. April 2005. doi:10.1038/ng1531. PMID 15765097.
- ↑ "The epigenetic modifier JMJD6 is amplified in mammary tumors and cooperates with c-Myc to enhance cellular transformation, tumor progression, and metastasis". Clinical Epigenetics 8 (38): 38. Apr 2016. doi:10.1186/s13148-016-0205-6. PMID 27081402.
- ↑ "Histone H4 lysine 16 acetylation regulates cellular lifespan". Nature 459 (7248): 802–807. June 2009. doi:10.1038/nature08085. PMID 19516333. Bibcode: 2009Natur.459..802D.
- ↑ "The Polycomb group protein EZH2 directly controls DNA methylation". Nature 439 (7078): 871–874. February 2006. doi:10.1038/nature04431. PMID 16357870. Bibcode: 2006Natur.439..871V.
- ↑ "Histone deacetylase inhibitor selectively induces p21WAF1 expression and gene-associated histone acetylation". Proceedings of the National Academy of Sciences of the United States of America 97 (18): 10014–10019. August 2000. doi:10.1073/pnas.180316197. PMID 10954755. Bibcode: 2000PNAS...9710014R.
- ↑ "Cancer research: Open ambition". Nature 488 (7410): 148–150. August 2012. doi:10.1038/488148a. PMID 22874946. Bibcode: 2012Natur.488..148M.
- ↑ 28.0 28.1 "Epigenetic regulation of the human p53 gene promoter by the CTCF transcription factor in transformed cell lines". Oncogene 29 (15): 2217–2227. April 2010. doi:10.1038/onc.2009.509. PMID 20101205.
- ↑ "Immunopositivity for histone macroH2A1 isoforms marks steatosis-associated hepatocellular carcinoma". PLOS ONE 8 (1): e54458. 2013. doi:10.1371/journal.pone.0054458. PMID 23372727. Bibcode: 2013PLoSO...854458R.
- ↑ "A truncating mutation of HDAC2 in human cancers confers resistance to histone deacetylase inhibition". Nature Genetics 38 (5): 566–569. May 2006. doi:10.1038/ng1773. PMID 16642021.
- ↑ Verhelst, Sigrid; Van Puyvelde, Bart; Willems, Sander; Daled, Simon; Cornelis, Senne; Corveleyn, Laura; Willems, Ewoud; Deforce, Dieter et al. (2022-01-24). "A large scale mass spectrometry-based histone screening for assessing epigenetic developmental toxicity" (in en). Scientific Reports 12 (1): 1256. doi:10.1038/s41598-022-05268-x. ISSN 2045-2322. PMID 35075221. Bibcode: 2022NatSR..12.1256V. https://www.nature.com/articles/s41598-022-05268-x.
- ↑ "Crosstalk between histone modifications during the DNA damage response". Trends in Cell Biology 19 (5): 207–217. May 2009. doi:10.1016/j.tcb.2009.03.001. PMID 19342239.
- ↑ "Most mammalian mRNAs are conserved targets of microRNAs". Genome Research 19 (1): 92–105. January 2009. doi:10.1101/gr.082701.108. PMID 18955434.
- ↑ "Specific activation of microRNA-127 with downregulation of the proto-oncogene BCL6 by chromatin-modifying drugs in human cancer cells". Cancer Cell 9 (6): 435–443. June 2006. doi:10.1016/j.ccr.2006.04.020. PMID 16766263.
- ↑ "Genetic unmasking of an epigenetically silenced microRNA in human cancer cells". Cancer Research 67 (4): 1424–1429. February 2007. doi:10.1158/0008-5472.CAN-06-4218. PMID 17308079.
- ↑ 36.0 36.1 "Disruption of CTCF at the miR-125b1 locus in gynecological cancers". BMC Cancer 12: 40. January 2012. doi:10.1186/1471-2407-12-40. PMID 22277129.
- ↑ "miRNA gene promoters are frequent targets of aberrant DNA methylation in human breast cancer". PLOS ONE 8 (1): e54398. 2013. doi:10.1371/journal.pone.0054398. PMID 23342147. Bibcode: 2013PLoSO...854398V.
- ↑ 38.0 38.1 "Metabolic recoding of epigenetics in cancer". Cancer Communications 38 (1): 25. May 2018. doi:10.1186/s40880-018-0302-3. PMID 29784032.
- ↑ "DNA damage responses: mechanisms and roles in human disease: 2007 G.H.A. Clowes Memorial Award Lecture". Molecular Cancer Research 6 (4): 517–524. April 2008. doi:10.1158/1541-7786.MCR-08-0020. PMID 18403632.
- ↑ 40.0 40.1 "Chapter 16: DNA Damage, DNA Repair and Cancer". New Research Directions in DNA Repair. BoD – Books on Demand. 2013. p. 413. ISBN 978-953-51-1114-6.
- ↑ 41.0 41.1 "Double strand breaks can initiate gene silencing and SIRT1-dependent onset of DNA methylation in an exogenous promoter CpG island". PLOS Genetics 4 (8): e1000155. August 2008. doi:10.1371/journal.pgen.1000155. PMID 18704159.
- ↑ 42.0 42.1 "DNA damage, homology-directed repair, and DNA methylation". PLOS Genetics 3 (7): e110. July 2007. doi:10.1371/journal.pgen.0030110. PMID 17616978.
- ↑ "Hereditary and familial colon cancer". Gastroenterology 138 (6): 2044–2058. June 2010. doi:10.1053/j.gastro.2010.01.054. PMID 20420945.
- ↑ "miRNA response to DNA damage". Trends in Biochemical Sciences 36 (9): 478–484. September 2011. doi:10.1016/j.tibs.2011.06.002. PMID 21741842.
- ↑ "MicroRNAs in the DNA Damage/Repair Network and Cancer". International Journal of Genomics 2014: 820248. 2014. doi:10.1155/2014/820248. PMID 24616890.
- ↑ 46.0 46.1 "Epigenetics Offer New Horizons for Colorectal Cancer Prevention". Current Colorectal Cancer Reports 8 (1): 66–81. March 2012. doi:10.1007/s11888-011-0116-z. PMID 22389639.
- ↑ 47.0 47.1 "Modulation of mismatch repair and genomic stability by miR-155". Proceedings of the National Academy of Sciences of the United States of America 107 (15): 6982–6987. April 2010. doi:10.1073/pnas.1002472107. PMID 20351277. Bibcode: 2010PNAS..107.6982V.
- ↑ 48.0 48.1 "Immunohistochemical analysis reveals high frequency of PMS2 defects in colorectal cancer". Gastroenterology 128 (5): 1160–1171. May 2005. doi:10.1053/j.gastro.2005.01.056. PMID 15887099.
- ↑ 49.0 49.1 49.2 "miR-181d: a predictive glioblastoma biomarker that downregulates MGMT expression". Neuro-Oncology 14 (6): 712–719. June 2012. doi:10.1093/neuonc/nos089. PMID 22570426.
- ↑ "O6-Methylguanine DNA methyltransferase protein expression in tumor cells predicts outcome of temozolomide therapy in glioblastoma patients". Neuro-Oncology 12 (1): 28–36. January 2010. doi:10.1093/neuonc/nop003. PMID 20150365.
- ↑ 51.0 51.1 "Downregulation of HMGA-targeting microRNAs has a critical role in human pituitary tumorigenesis". Oncogene 31 (34): 3857–3865. August 2012. doi:10.1038/onc.2011.557. PMID 22139073.
- ↑ "Nuclear phosphoproteins HMGA and their relationship with chromatin structure and cancer". FEBS Letters 574 (1–3): 1–8. September 2004. doi:10.1016/j.febslet.2004.08.013. PMID 15358530.
- ↑ "The HMG-I oncogene causes highly penetrant, aggressive lymphoid malignancy in transgenic mice and is overexpressed in human leukemia". Cancer Research 64 (10): 3371–3375. May 2004. doi:10.1158/0008-5472.CAN-04-0044. PMID 15150086.
- ↑ "Negative regulation of BRCA1 gene expression by HMGA1 proteins accounts for the reduced BRCA1 protein levels in sporadic breast carcinoma". Molecular and Cellular Biology 23 (7): 2225–2238. April 2003. doi:10.1128/MCB.23.7.2225-2238.2003. PMID 12640109.
- ↑ "High mobility group A2 protein and its derivatives bind a specific region of the promoter of DNA repair gene ERCC1 and modulate its activity". Nucleic Acids Research 31 (23): 6841–6851. December 2003. doi:10.1093/nar/gkg884. PMID 14627817.
- ↑ 56.0 56.1 56.2 56.3 "Deficient expression of DNA repair enzymes in early progression to sporadic colon cancer". Genome Integrity 3 (1): 3. April 2012. doi:10.1186/2041-9414-3-3. PMID 22494821.
- ↑ "miRNAs and cancer: an epigenetics view". Molecular Aspects of Medicine 34 (4): 863–874. 2013. doi:10.1016/j.mam.2012.06.005. PMID 22771542.
- ↑ "Histone deacetylases mediate the silencing of miR-15a, miR-16, and miR-29b in chronic lymphocytic leukemia". Blood 119 (5): 1162–1172. February 2012. doi:10.1182/blood-2011-05-351510. PMID 22096249.
- ↑ Human DNA Repair Genes, 15 April 2014, MD Anderson Cancer Center, University of Texas
- ↑ "MicroRNA-182-5p targets a network of genes involved in DNA repair". RNA 19 (2): 230–242. February 2013. doi:10.1261/rna.034926.112. PMID 23249749.
- ↑ "Epigenetic screen of human DNA repair genes identifies aberrant promoter methylation of NEIL1 in head and neck squamous cell carcinoma". Oncogene 31 (49): 5108–5116. December 2012. doi:10.1038/onc.2011.660. PMID 22286769.
- ↑ "Modulation of DNA end joining by nuclear proteins". The Journal of Biological Chemistry 280 (36): 31442–31449. September 2005. doi:10.1074/jbc.M503776200. PMID 16012167.
- ↑ "Overexpression and hypomethylation of flap endonuclease 1 gene in breast and other cancers". Molecular Cancer Research 6 (11): 1710–1717. November 2008. doi:10.1158/1541-7786.MCR-08-0269. PMID 19010819.
- ↑ "Flap endonuclease 1 is overexpressed in prostate cancer and is associated with a high Gleason score". BJU International 98 (2): 445–451. August 2006. doi:10.1111/j.1464-410X.2006.06224.x. PMID 16879693.
- ↑ "Identification of gastric cancer-related genes using a cDNA microarray containing novel expressed sequence tags expressed in gastric cancer cells". Clinical Cancer Research 11 (2 Pt 1): 473–482. January 2005. doi:10.1158/1078-0432.473.11.2. PMID 15701830.
- ↑ "Flap endonuclease 1 is a promising candidate biomarker in gastric cancer and is involved in cell proliferation and apoptosis". International Journal of Molecular Medicine 33 (5): 1268–1274. May 2014. doi:10.3892/ijmm.2014.1682. PMID 24590400.
- ↑ "Genome-wide analysis of gene expression in neuroblastomas detected by mass screening". Cancer Letters 225 (1): 111–120. July 2005. doi:10.1016/j.canlet.2004.10.035. PMID 15922863. https://hal.archives-ouvertes.fr/hal-00157917/file/Cancer_Letters_2004.pdf.
- ↑ "Exploration of global gene expression patterns in pancreatic adenocarcinoma using cDNA microarrays". The American Journal of Pathology 162 (4): 1151–1162. April 2003. doi:10.1016/S0002-9440(10)63911-9. PMID 12651607.
- ↑ "Increased expression and no mutation of the Flap endonuclease (FEN1) gene in human lung cancer". Oncogene 22 (46): 7243–7246. October 2003. doi:10.1038/sj.onc.1206977. PMID 14562054.
- ↑ "Hypomethylation of ETS transcription factor binding sites and upregulation of PARP1 expression in endometrial cancer". BioMed Research International 2013: 946268. 2013. doi:10.1155/2013/946268. PMID 23762867.
- ↑ "Poly (ADP-ribose) polymerase 1 transcriptional regulation: a novel crosstalk between histone modification H3K9ac and ETS1 motif hypomethylation in BRCA1-mutated ovarian cancer". Oncotarget 5 (1): 291–297. January 2014. doi:10.18632/oncotarget.1549. PMID 24448423.
- ↑ "Promoter hypomethylation, especially around the E26 transformation-specific motif, and increased expression of poly (ADP-ribose) polymerase 1 in BRCA-mutated serous ovarian cancer". BMC Cancer 13: 90. February 2013. doi:10.1186/1471-2407-13-90. PMID 23442605.
- ↑ "Elevated levels of mutation in multiple tissues of mice deficient in the DNA mismatch repair gene Pms2". Proceedings of the National Academy of Sciences of the United States of America 94 (7): 3122–3127. April 1997. doi:10.1073/pnas.94.7.3122. PMID 9096356. Bibcode: 1997PNAS...94.3122N.
- ↑ "Differing patterns of genetic instability in mice deficient in the mismatch repair genes Pms2, Mlh1, Msh2, Msh3 and Msh6". Carcinogenesis 27 (12): 2402–2408. December 2006. doi:10.1093/carcin/bgl079. PMID 16728433.
- ↑ "Disruption of Brca2 increases the spontaneous mutation rate in vivo: synergism with ionizing radiation". EMBO Reports 3 (3): 255–260. March 2002. doi:10.1093/embo-reports/kvf037. PMID 11850397.
- ↑ "O(6)-methylguanine methyltransferase in colorectal cancers: detection of mutations, loss of expression, and weak association with G:C>A:T transitions". Gut 54 (6): 797–802. June 2005. doi:10.1136/gut.2004.059535. PMID 15888787.
- ↑ "MGMT promoter methylation and field defect in sporadic colorectal cancer". Journal of the National Cancer Institute 97 (18): 1330–1338. September 2005. doi:10.1093/jnci/dji275. PMID 16174854.
- ↑ 78.0 78.1 "Promoter methylation status of hMLH1, hMSH2, and MGMT genes in colorectal cancer associated with adenoma-carcinoma sequence". Langenbeck's Archives of Surgery 396 (7): 1017–1026. October 2011. doi:10.1007/s00423-011-0812-9. PMID 21706233.
- ↑ "Methylation tolerance due to an O6-methylguanine DNA methyltransferase (MGMT) field defect in the colonic mucosa: an initiating step in the development of mismatch repair-deficient colorectal cancers". Gut 59 (11): 1516–1526. November 2010. doi:10.1136/gut.2009.194787. PMID 20947886.
- ↑ "Frequent hypermethylation of DAPK, RARbeta, MGMT, RASSF1A and FHIT in laryngeal squamous cell carcinomas and adjacent normal mucosa". Oral Oncology 47 (2): 104–107. February 2011. doi:10.1016/j.oraloncology.2010.11.006. PMID 21147548.
- ↑ "Increased microsatellite instability and epigenetic inactivation of the hMLH1 gene in head and neck squamous cell carcinoma". Otolaryngology–Head and Neck Surgery 141 (4): 484–490. October 2009. doi:10.1016/j.otohns.2009.07.007. PMID 19786217.
- ↑ "Head and neck squamous cell carcinoma: mismatch repair immunohistochemistry and promoter hypermethylation of hMLH1 gene". American Journal of Otolaryngology 32 (6): 528–536. 2011. doi:10.1016/j.amjoto.2010.11.005. PMID 21353335.
- ↑ "Promoter hypermethylation of multiple genes in early gastric adenocarcinoma and precancerous lesions". Human Pathology 40 (11): 1534–1542. November 2009. doi:10.1016/j.humpath.2009.01.029. PMID 19695681.
- ↑ "Promoter methylation status of DNA repair gene (hMLH1) in gastric carcinoma patients of the Kashmir valley". Asian Pacific Journal of Cancer Prevention 13 (8): 4177–4181. 2012. doi:10.7314/APJCP.2012.13.8.4177. PMID 23098428.
- ↑ 85.0 85.1 "Helicobacter pylori severely reduces expression of DNA repair proteins PMS2 and ERCC1 in gastritis and gastric cancer". DNA Repair 89: 102836. May 2020. doi:10.1016/j.dnarep.2020.102836. PMID 32143126.
- ↑ "Role of epigenetic alterations in the pathogenesis of Barrett's esophagus and esophageal adenocarcinoma". International Journal of Clinical and Experimental Pathology 5 (5): 382–396. 2012. PMID 22808291.
- ↑ "Genomic sequencing in cancer". Cancer Letters 340 (2): 161–170. November 2013. doi:10.1016/j.canlet.2012.11.004. PMID 23178448.
- ↑ "Analysis of genetic inheritance in a family quartet by whole-genome sequencing". Science 328 (5978): 636–639. April 2010. doi:10.1126/science.1186802. PMID 20220176. Bibcode: 2010Sci...328..636R.
- ↑ "Estimating the human mutation rate using autozygosity in a founder population". Nature Genetics 44 (11): 1277–1281. November 2012. doi:10.1038/ng.2418. PMID 23001126.
- ↑ "Rates and fitness consequences of new mutations in humans". Genetics 190 (2): 295–304. February 2012. doi:10.1534/genetics.111.134668. PMID 22345605.
- ↑ "Aging as accelerated accumulation of somatic variants: whole-genome sequencing of centenarian and middle-aged monozygotic twin pairs". Twin Research and Human Genetics 16 (6): 1026–1032. December 2013. doi:10.1017/thg.2013.73. PMID 24182360.
- ↑ "ATM-dependent chromatin changes silence transcription in cis to DNA double-strand breaks". Cell 141 (6): 970–981. June 2010. doi:10.1016/j.cell.2010.04.038. PMID 20550933.
- ↑ "Targeted DNA methylation by homology-directed repair in mammalian cells. Transcription reshapes methylation on the repaired gene". Nucleic Acids Research 42 (2): 804–821. January 2014. doi:10.1093/nar/gkt920. PMID 24137009.
- ↑ "A DNA methylation fingerprint of 1628 human samples". Genome Research 22 (2): 407–419. February 2012. doi:10.1101/gr.119867.110. PMID 21613409.
- ↑ "Genetic toxicities of human teratogens". Mutation Research 396 (1–2): 9–43. December 1997. doi:10.1016/S0027-5107(97)00173-5. PMID 9434858. https://zenodo.org/record/1259667.
- ↑ "Association of valproate-induced teratogenesis with histone deacetylase inhibition in vivo". FASEB Journal 19 (9): 1166–1168. July 2005. doi:10.1096/fj.04-3425fje. PMID 15901671.
- ↑ "Does thalidomide cause second generation birth defects?". Drug Safety 19 (5): 339–341. November 1998. doi:10.2165/00002018-199819050-00001. PMID 9825947.
- ↑ "Paternal exposures: impact on reproductive and developmental outcome. An overview". Pharmacology, Biochemistry, and Behavior 55 (4): 691–700. December 1996. doi:10.1016/S0091-3057(96)00286-9. PMID 8981601.
- ↑ "Vidaza (azacitidine for injectable suspension) package insert". Pharmion Corporation. U.S. Food and Drug Administration. 18 May 2004. http://www.fda.gov/cder/foi/label/2004/050794lbl.pdf.
- ↑ "Influence of morphine exposure during adolescence on the sexual maturation of male rats and the development of their offspring". The Journal of Pharmacology and Experimental Therapeutics 256 (3): 1086–1093. March 1991. PMID 2005573.
- ↑ "Adverse effects of the model environmental estrogen diethylstilbestrol are transmitted to subsequent generations". Endocrinology 147 (6 Suppl): S11–S17. June 2006. doi:10.1210/en.2005-1164. PMID 16690809.
- ↑ 102.0 102.1 "Epigenetic alterations in ultraviolet radiation-induced skin carcinogenesis: interaction of bioactive dietary components on epigenetic targets". Photochemistry and Photobiology 88 (5): 1066–1074. September 2012. doi:10.1111/j.1751-1097.2011.01020.x. PMID 22017262.
- ↑ "Tackling malignant melanoma epigenetically: histone lysine methylation". Clinical Epigenetics 10 (1): 145. November 2018. doi:10.1186/s13148-018-0583-z. PMID 30466474.
- ↑ 104.0 104.1 "Next generation sequencing of prostate cancer from a patient identifies a deficiency of methylthioadenosine phosphorylase, an exploitable tumor target". Molecular Cancer Therapeutics 11 (3): 775–783. March 2012. doi:10.1158/1535-7163.MCT-11-0826. PMID 22252602.
- ↑ 105.0 105.1 "Epigenetic changes in prostate cancer: implication for diagnosis and treatment". Journal of the National Cancer Institute 97 (2): 103–115. January 2005. doi:10.1093/jnci/dji010. PMID 15657340.
- ↑ 106.0 106.1 "Molecular alterations in prostate cancer as diagnostic, prognostic, and therapeutic targets". Advances in Anatomic Pathology 15 (6): 319–331. November 2008. doi:10.1097/PAP.0b013e31818a5c19. PMID 18948763.
- ↑ "Changes in prostate gene expression in men undergoing an intensive nutrition and lifestyle intervention". Proceedings of the National Academy of Sciences of the United States of America 105 (24): 8369–8374. June 2008. doi:10.1073/pnas.0803080105. PMID 18559852. Bibcode: 2008PNAS..105.8369O.
- ↑ 108.0 108.1 108.2 108.3 108.4 108.5 108.6 108.7 "Methylation of HPV16 genome CpG sites is associated with cervix precancer and cancer". Gynecologic Oncology 121 (1): 59–63. April 2011. doi:10.1016/j.ygyno.2011.01.013. PMID 21306759.
- ↑ "Mixed lineage leukemia: versatile player in epigenetics and human disease". The FEBS Journal 277 (8): 1789. April 2010. doi:10.1111/j.1742-4658.2010.07605.x. PMID 20236314.
- ↑ "Cancer epigenetics: Moving forward". PLOS Genetics 14 (6): e1007362. June 2018. doi:10.1371/journal.pgen.1007362. PMID 29879107.
- ↑ "Electromagnetic fields may cause leukaemia in children" (in en). The Lancet 357 (9258): 777. March 2001. doi:10.1016/S0140-6736(05)71207-1.
- ↑ "Soft Tissue Sarcoma". National Cancer Institute. National Institutes of Health, U.S. Department of Health and Human Services. January 1980. http://www.cancer.gov/research/progress/snapshots/sarcoma.
- ↑ "Epigenetic and epigenomic mechanisms shape sarcoma and other mesenchymal tumor pathogenesis". Epigenomics 3 (6): 715–732. December 2011. doi:10.2217/epi.11.93. PMID 22126291.
- ↑ "EZH2 is a mediator of EWS/FLI1 driven tumor growth and metastasis blocking endothelial and neuro-ectodermal differentiation". Proceedings of the National Academy of Sciences of the United States of America 106 (13): 5324–5329. March 2009. doi:10.1073/pnas.0810759106. PMID 19289832. Bibcode: 2009PNAS..106.5324R.
- ↑ "Lysine-specific demethylase 1 (LSD1/KDM1A/AOF2/BHC110) is expressed and is an epigenetic drug target in chondrosarcoma, Ewing's sarcoma, osteosarcoma, and rhabdomyosarcoma". Human Pathology 43 (8): 1300–1307. August 2012. doi:10.1016/j.humpath.2011.10.010. PMID 22245111.
- ↑ Tam, Kit W.; Zhang, Wei; Soh, Junichi; Stastny, Victor; Chen, Min; Sun, Han; Thu, Kelsie; Rios, Jonathan J. et al. (2013-11-01). "CDKN2A/p16 Inactivation Mechanisms and Their Relationship to Smoke Exposure and Molecular Features in Non–Small-Cell Lung Cancer" (in en). Journal of Thoracic Oncology 8 (11): 1378–1388. doi:10.1097/JTO.0b013e3182a46c0c. ISSN 1556-0864. PMID 24077454.
- ↑ Hong, Runyu; Liu, Wenke; Fenyö, David (2021). "Predicting and Visualizing STK11 Mutation in Lung Adenocarcinoma Histopathology Slides Using Deep Learning". doi:10.1101/2020.02.20.956557. http://dx.doi.org/10.1101/2020.02.20.956557.
- ↑ Ahmad, Aamir (2015). Lung Cancer and Personalized Medicine: Novel Therapies and Clinical Management. Springer International Publishing. ISBN 978-3-319-24932-2. OCLC 1113687835. http://worldcat.org/oclc/1113687835.
- ↑ Iwakawa, Reika; Kohno, Takashi; Anami, Yoichi; Noguchi, Masayuki; Suzuki, Kenji; Matsuno, Yoshihiro; Mishima, Kazuhiko; Nishikawa, Ryo et al. (2008-06-15). "Association of p16 Homozygous Deletions with Clinicopathologic Characteristics and EGFR/KRAS/p53 Mutations in Lung Adenocarcinoma". Clinical Cancer Research 14 (12): 3746–3753. doi:10.1158/1078-0432.ccr-07-4552. ISSN 1078-0432. PMID 18559592. http://dx.doi.org/10.1158/1078-0432.ccr-07-4552.
- ↑ Fountain, J. W.; Giendening, J. M.; Flores, J. F. (1994-09-01). "Characterization of the p16 gene in the mouse: Evidence for a large gene family" (in English). American Journal of Human Genetics 55 (Suppl.3). https://www.osti.gov/biblio/133832.
- ↑ "Generating mutations but providing chemosensitivity: the role of O6-methylguanine DNA methyltransferase in human cancer". Oncogene 23 (1): 1–8. January 2004. doi:10.1038/sj.onc.1207316. PMID 14712205.
- ↑ "Inactivation of the DNA-repair gene MGMT and the clinical response of gliomas to alkylating agents". The New England Journal of Medicine 343 (19): 1350–1354. November 2000. doi:10.1056/NEJM200011093431901. PMID 11070098.
- ↑ "MGMT gene silencing and benefit from temozolomide in glioblastoma". The New England Journal of Medicine 352 (10): 997–1003. March 2005. doi:10.1056/NEJMoa043331. PMID 15758010. https://serval.unil.ch/resource/serval:BIB_59DF1F31757A.P001/REF.pdf.
- ↑ "Hypermethylation of the DNA repair gene O(6)-methylguanine DNA methyltransferase and survival of patients with diffuse large B-cell lymphoma". Journal of the National Cancer Institute 94 (1): 26–32. January 2002. doi:10.1093/jnci/94.1.26. PMID 11773279.
- ↑ "Epigenetics as a mechanism driving polygenic clinical drug resistance". British Journal of Cancer 94 (8): 1087–1092. April 2006. doi:10.1038/sj.bjc.6603024. PMID 16495912.
- ↑ "DNA methylation markers and early recurrence in stage I lung cancer". The New England Journal of Medicine 358 (11): 1118–1128. March 2008. doi:10.1056/NEJMoa0706550. PMID 18337602.
- ↑ 127.0 127.1 "Potential role of HDAC inhibitors in cancer therapy: insights into oral squamous cell carcinoma". Oral Oncology 46 (5): 323–329. May 2010. doi:10.1016/j.oraloncology.2010.01.009. PMID 20207580.
- ↑ "Prostate cancer chemopreventive activity of phenethyl isothiocyanate through epigenetic regulation (review)". International Journal of Oncology 37 (3): 533–539. September 2010. doi:10.3892/ijo_00000702. PMID 20664922.
- ↑ "Targeting Cancer with Epi-Drugs: A Precision Medicine Perspective". Current Pharmaceutical Biotechnology 17 (10): 856–865. 2016-05-27. doi:10.2174/1381612822666160527154757. PMID 27229488.
- ↑ "Cancer treatment of the future: inhibitors of histone methyltransferases". The International Journal of Biochemistry & Cell Biology 41 (1): 4–11. January 2009. doi:10.1016/j.biocel.2008.07.024. PMID 18773966.
- ↑ "A pilot pharmacokinetic study of oral azacitidine". Leukemia 22 (9): 1680–1684. September 2008. doi:10.1038/leu.2008.145. PMID 18548103.
- ↑ "Demethylating agents in myeloid malignancies". Current Opinion in Oncology 20 (6): 705–710. November 2008. doi:10.1097/CCO.0b013e328313699c. PMID 18841054.
- ↑ "Activity of decitabine, a hypomethylating agent, in chronic myelomonocytic leukemia". Cancer 109 (4): 713–717. February 2007. doi:10.1002/cncr.22457. PMID 17219444.
- ↑ "Feasibility of allo-SCT after hypomethylating therapy with decitabine for myelodysplastic syndrome". Bone Marrow Transplantation 43 (11): 839–843. June 2009. doi:10.1038/bmt.2008.400. PMID 19151791.
- ↑ "Hypomethylating drugs convert HA-1-negative solid tumors into targets for stem cell-based immunotherapy". Blood 113 (12): 2715–2722. March 2009. doi:10.1182/blood-2008-05-158956. PMID 19096014.
- ↑ "Efficacy of azacitidine compared with that of conventional care regimens in the treatment of higher-risk myelodysplastic syndromes: a randomised, open-label, phase III study". The Lancet. Oncology 10 (3): 223–232. March 2009. doi:10.1016/S1470-2045(09)70003-8. PMID 19230772.
- ↑ "Phase 2 trial of oral vorinostat (suberoylanilide hydroxamic acid, SAHA) for refractory cutaneous T-cell lymphoma (CTCL)". Blood 109 (1): 31–39. January 2007. doi:10.1182/blood-2006-06-025999. PMID 16960145.
- ↑ "Phase IIb multicenter trial of vorinostat in patients with persistent, progressive, or treatment refractory cutaneous T-cell lymphoma". Journal of Clinical Oncology 25 (21): 3109–3115. July 2007. doi:10.1200/JCO.2006.10.2434. PMID 17577020.
- ↑ "Synergy of demethylation and histone deacetylase inhibition in the re-expression of genes silenced in cancer". Nature Genetics 21 (1): 103–107. January 1999. doi:10.1038/5047. PMID 9916800.
- ↑ "New Drug Application: Panobinostat". Center for Drug Evaluation and Research. U.S. Food and Drug Administration. 14 January 2015. http://www.accessdata.fda.gov/drugsatfda_docs/nda/2015/205353Orig1s000MedR.pdf.
- ↑ "Toward the development of potent and selective bisubstrate inhibitors of protein arginine methyltransferases". Bioorganic & Medicinal Chemistry Letters 20 (7): 2103–2105. April 2010. doi:10.1016/j.bmcl.2010.02.069. PMID 20219369.
- ↑ "Chemopreventive Property of a Soybean Peptide (Lunasin) That Binds to Deacetylated Histones and Inhibits Acetylation". Cancer Research 61. October 15, 2001. http://cancerres.aacrjournals.org/content/61/20/7473.long.
- ↑ Brown, Robert; Strathdee, Gordon (2002-04-01). "Epigenomics and epigenetic therapy of cancer" (in en). Trends in Molecular Medicine 8 (4): S43–S48. doi:10.1016/S1471-4914(02)02314-6. ISSN 1471-4914. PMID 11927287. https://www.sciencedirect.com/science/article/pii/S1471491402023146.
- ↑ Egger, Gerda; Liang, Gangning; Aparicio, Ana; Jones, Peter A. (May 2004). "Epigenetics in human disease and prospects for epigenetic therapy" (in en). Nature 429 (6990): 457–463. doi:10.1038/nature02625. ISSN 1476-4687. PMID 15164071. Bibcode: 2004Natur.429..457E. https://www.nature.com/articles/nature02625.
- ↑ Song, Sang-Hyun; Han, Sae-Won; Bang, Yung-Jue (2011-12-01). "Epigenetic-Based Therapies in Cancer" (in en). Drugs 71 (18): 2391–2403. doi:10.2165/11596690-000000000-00000. ISSN 1179-1950. PMID 22141383. https://doi.org/10.2165/11596690-000000000-00000.
- ↑ Saleem, Mohammad (May 2015). "Epigenetic Therapy for Caner". Pak. J. Pharm. Sci 28 (3): 1023–1032. https://www.researchgate.net/publication/273648245.
- ↑ 147.0 147.1 "Error: no
|title=
specified when using {{Cite web}}". https://aacrjournals.org/clincancerres/article/13/6/1634/195851/DNA-Methylation-as-a-Therapeutic-Target-in-Cancer. - ↑ Kantarjian, Hagop; Issa, Jean-Pierre J.; Rosenfeld, Craig S.; Bennett, John M.; Albitar, Maher; DiPersio, John; Klimek, Virginia; Slack, James et al. (2006-04-15). "Decitabine improves patient outcomes in myelodysplastic syndromes: Results of a phase III randomized study" (in en). Cancer 106 (8): 1794–1803. doi:10.1002/cncr.21792. ISSN 0008-543X. PMID 16532500.
![]() | Original source: https://en.wikipedia.org/wiki/Cancer epigenetics.
Read more |