Chemistry:Hydrogel
A hydrogel is a biphasic material, a mixture of porous, permeable solids and at least 10% by weight or volume of interstitial fluid composed completely or mainly by water.[1][2] In hydrogels the porous permeable solid is a water insoluble three dimensional network of natural or synthetic polymers and a fluid, having absorbed a large amount of water or biological fluids.[2][3][4][5] These properties underpin several applications, especially in the biomedical area. Many hydrogels are synthetic, but some are derived from nature.[6][7] The term 'hydrogel' was coined in 1894.[8]
Chemistry
Classification
The crosslinks which bond the polymers of a hydrogel fall under two general categories: physical hydrogels and chemical hydrogels. Chemical hydrogels have covalent cross-linking bonds, whereas physical hydrogels have non-covalent bonds.[citation needed] Chemical hydrogels can result in strong reversible or irreversible gels due to the covalent bonding.[9] Chemical hydrogels that contain reversible covalent cross-linking bonds such as hydrogels of thiomers being cross-linked via disulfide bonds are non-toxic and are used in numerous medicinal products.[10][11][12] Physical hydrogels usually have high biocompatibility, are not toxic, and are also easily reversible by simply changing an external stimulus such as pH, ion concentration (alginate) or temperature (gelatine); they are also used for medical applications.[13][14][15][16][17] Physical crosslinks consist of hydrogen bonds, hydrophobic interactions, and chain entanglements (among others). A hydrogel generated through the use of physical crosslinks is sometimes called a 'reversible' hydrogel.[13] Chemical crosslinks consist of covalent bonds between polymer strands. Hydrogels generated in this manner are sometimes called 'permanent' hydrogels.
Hydrogels are prepared using a variety of polymeric materials, which can be divided broadly into two categories according to their origin: natural or synthetic polymers. Natural polymers for hydrogel preparation include hyaluronic acid, chitosan, heparin, alginate, gelatin and fibrin.[18] Common synthetic polymers include polyvinyl alcohol, polyethylene glycol, sodium polyacrylate, acrylate polymers and copolymers thereof.[6] Whereas natural hydrogels are usually non-toxic, and often provides other advantages for medical use, such as biocompatibility, biodegradability, antibiotic/antifungal effect and improve regeneration of nearby tissue, their stability and strength is usually much lower than synthetic hydrogels.[19] There are also synthetic hydrogels than can be used for medical applications, such as polyethylene glycol (PEG), polyacrylate, and polyvinylpyrrolidone (PVP).[20]
Preparation
There are two suggested mechanisms behind physical hydrogel formation, the first one being the gelation of nanofibrous peptide assemblies, usually observed for oligopeptide precursors. The precursors self-assemble into fibers, tapes, tubes, or ribbons that entangle to form non-covalent cross-links. The second mechanism involves non-covalent interactions of cross-linked domains that are separated by water-soluble linkers, and this is usually observed in longer multi-domain structures.[21] Tuning of the supramolecular interactions to produce a self-supporting network that does not precipitate, and is also able to immobilize water which is vital for to gel formation. Most oligopeptide hydrogels have a β-sheet structure, and assemble to form fibers, although α-helical peptides have also been reported.[22][23] The typical mechanism of gelation involves the oligopeptide precursors self-assemble into fibers that become elongated, and entangle to form cross-linked gels.
One notable method of initiating a polymerization fuving involves the use of light as a stimulus. In this method, photoinitiators, compounds that cleave from the absorption of photons, are added to the precursor solution which will become the hydrogel. When the precursor solution is exposed to a concentrated source of light, usually ultraviolet irradiation, the photoinitiators will cleave and form free radicals, which will begin a polymerization reaction that forms crosslinks between polymer strands. This reaction will cease if the light source is removed, allowing the amount of crosslinks formed in the hydrogel to be controlled.[24] The properties of a hydrogel are highly dependent on the type and quantity of its crosslinks, making photopolymerization a popular choice for fine-tuning hydrogels. This technique has seen considerable use in cell and tissue engineering applications due to the ability to inject or mold a precursor solution loaded with cells into a wound site, then solidify it in situ.[25][24]
Physically crosslinked hydrogels can be prepared by different methods depending on the nature of the crosslink involved. Polyvinyl alcohol hydrogels are usually produced by the freeze-thawed technique. In this, the solution is frozen for a few hours, then thawed at room temperature, and the cycle is repeated until a strong and stable hydrogel is formed.[26] Alginate hydrogels are formed by ionic interactions between alginate and double-charged cations. A salt, usually calcium chloride, is dissolved into an aqueous sodium alginate solution, that causes the calcium ions to create ionic bonds between alginate chains.[27] Gelatin hydrogels are formed by temperature change. A water solution of gelatin forms an hydrogel at temperatures below 37–35 °C, as Van der Waals interactions between collagen fibers become stronger than thermal molecular vibrations.[28]
Peptides based hydrogels
Peptides based hydrogels possess exceptional biocompatibility and biodegradability qualities, giving rise to their wide use of applications, particularly in biomedicine;[2] as such, their physical properties can be fine-tuned in order to maximise their use.[2] Methods to do this are: modulation of the amino acid sequence, pH, chirality, and increasing the number of aromatic residues.[29] The order of amino acids within the sequence is crucial for gelation, as has been shown many times. In one example, a short peptide sequence Fmoc-Phe-Gly readily formed a hydrogel, whereas Fmoc-Gly-Phe failed to do so as a result of the two adjacent aromatic moieties being moved, hindering the aromatic interactions.[30][31] Altering the pH can also have similar effects, an example involved the use of the naphthalene (Nap) modified dipeptides Nap-Gly-Ala, and Nap- Ala-Gly, where a drop in pH induced gelation of the former, but led to crystallisation of the latter.[32] A controlled pH decrease method using glucono-δ-lactone (GdL), where the GdL is hydrolysed to gluconic acid in water is a recent strategy that has been developed as a way to form homogeneous and reproducible hydrogels.[33][34] The hydrolysis is slow, which allows for a uniform pH change, and thus resulting in reproducible homogenous gels. In addition to this, the desired pH can be achieved by altering the amount of GdL added. The use of GdL has been used various times for the hydrogelation of Fmoc and Nap-dipeptides.[33][34] In another direction, Morris et al reported the use of GdL as a ‘molecular trigger’ to predict and control the order of gelation.[35] Chirality also plays an essential role in gel formation, and even changing the chirality of a single amino acid from its natural L-amino acid to its unnatural D-amino acid can significantly impact the gelation properties, with the natural forms not forming gels.[36] Furthermore, aromatic interactions play a key role in hydrogel formation as a result of π- π stacking driving gelation, shown by many studies.[37][38]
Other
Hydrogels also possess a degree of flexibility very similar to natural tissue due to their significant water content. As responsive "smart materials", hydrogels can encapsulate chemical systems which upon stimulation by external factors such as a change of pH may cause specific compounds such as glucose to be liberated to the environment, in most cases by a gel–sol transition to the liquid state. Chemomechanical polymers are mostly also hydrogels, which upon stimulation change their volume and can serve as actuators or sensors.
Mechanical properties
Hydrogels have been investigated for diverse applications. By modifying the polymer concentration of a hydrogel (or conversely, the water concentration), the Young's modulus, shear modulus, and storage modulus can vary from 10 Pa to 3 MPa, a range of about five orders of magnitude.[40] A similar effect can be seen by altering the crosslinking concentration.[40] This much variability of the mechanical stiffness is why hydrogels are so appealing for biomedical applications, where it is vital for implants to match the mechanical properties of the surrounding tissues.[41] Characterizing the mechanical properties of hydrogels can be difficult especially due to the differences in mechanical behavior that hydrogels have in comparison to other traditional engineering materials. In addition to its rubber elasticity and viscoelasticity, hydrogels have an additional time dependent deformation mechanism which is dependent on fluid flow called poroelasticity. These properties are extremely important to consider while performing mechanical experiments. Some common mechanical testing experiments for hydrogels are tension, compression (confined or unconfined), indentation, shear rheometry or dynamic mechanical analysis.[40]
Hydrogels have two main regimes of mechanical properties: rubber elasticity and viscoelasticity:
Rubber elasticity
In the unswollen state, hydrogels can be modelled as highly crosslinked chemical gels, in which the system can be described as one continuous polymer network. In this case:
[math]\displaystyle{ G=N_{p}kT={\rho RT \over \overline{M}_{c}} }[/math]
where G is the shear modulus, k is the Boltzmann constant, T is temperature, Np is the number of polymer chains per unit volume, ρ is the density, R is the ideal gas constant, and [math]\displaystyle{ \overline{M}_{c} }[/math] is the (number) average molecular weight between two adjacent cross-linking points. [math]\displaystyle{ \overline{M}_{c} }[/math] can be calculated from the swell ratio, Q, which is relatively easy to test and measure.[40]
For the swollen state, a perfect gel network can be modeled as:[40]
[math]\displaystyle{ G_{\textrm{swollen}}=GQ^{-1/3} }[/math]
In a simple uniaxial extension or compression test, the true stress, [math]\displaystyle{ \sigma _{t} }[/math], and engineering stress, [math]\displaystyle{ \sigma _{e} }[/math], can be calculated as:
[math]\displaystyle{ \sigma _{t}=G_{\textrm{swollen}}\left ( \lambda ^{2}-\lambda ^{-1} \right ) }[/math]
[math]\displaystyle{ \sigma _{e}=G_{\textrm{swollen}}\left ( \lambda -\lambda ^{-2} \right ) }[/math]
where [math]\displaystyle{ \lambda =l_{\textrm{current}}/l_{\textrm{original}} }[/math] is the stretch.[40]
Viscoelasticity
For hydrogels, their elasticity comes from the solid polymer matrix while the viscosity originates from the polymer network mobility and the water and other components that make up the aqueous phase.[42] Viscoelastic properties of a hydrogel is highly dependent on the nature of the applied mechanical motion. Thus, the time dependence of these applied forces is extremely important for evaluating the viscoelasticity of the material.[43]
Physical models for viscoelasticity attempt to capture the elastic and viscous material properties of a material. In an elastic material, the stress is proportional to the strain while in a viscous material, the stress is proportional to the strain rate. The Maxwell model is one developed mathematical model for linear viscoelastic response. In this model, viscoelasticity is modeled analogous to an electrical circuit with a Hookean spring, that represents the Young's modulus, and a Newtonian dashpot that represents the viscosity. A material that exhibit properties described in this model is a Maxwell material. Another physical model used is called the Kelvin-Voigt Model and a material that follow this model is called a Kelvin–Voigt material.[44] In order to describe the time-dependent creep and stress-relaxation behavior of hydrogel, a variety of physical lumped parameter models can be used.[40] These modeling methods vary greatly and are extremely complex, so the empirical Prony Series description is commonly used to describe the viscoelastic behavior in hydrogels.[40]
In order to measure the time-dependent viscoelastic behavior of polymers dynamic mechanical analysis is often performed. Typically, in these measurements the one side of the hydrogel is subjected to a sinusoidal load in shear mode while the applied stress is measured with a stress transducer and the change in sample length is measured with a strain transducer.[43] One notation used to model the sinusoidal response to the periodic stress or strain is:
- [math]\displaystyle{ G = G' + iG'' }[/math]
in which G' is the real (elastic or storage) modulus, G" is the imaginary (viscous or loss) modulus.
Poroelasticity
Poroelasticity is a characteristic of materials related to the migration of solvent through a porous material and the concurrent deformation that occurs.[40] Poroelasticity in hydrated materials such as hydrogels occurs due to friction between the polymer and water as the water moves through the porous matrix upon compression. This causes a decrease in water pressure, which adds additional stress upon compression. Similar to viscoelasticity, this behavior is time dependent, thus poroelasticity is dependent on compression rate: a hydrogel shows softness upon slow compression, but fast compression makes the hydrogel stiffer. This phenomenon is due to the friction between the water and the porous matrix is proportional to the flow of water, which in turn is dependent on compression rate. Thus, a common way to measure poroelasticity is to do compression tests at varying compression rates.[45] Pore size is an important factor in influencing poroelasticity. The Kozeny–Carman equation has been used to predict pore size by relating the pressure drop to the difference in stress between two compression rates.[45]
Poroelasticity is described by several coupled equations, thus there are few mechanical tests that relate directly to the poroelastic behavior of the material, thus more complicated tests such as indentation testing, numerical or computational models are utilized. Numerical or computational methods attempt to simulate the three dimensional permeability of the hydrogel network.
Environmental response
The most commonly seen environmental sensitivity in hydrogels is a response to temperature.[46] Many polymers/hydrogels exhibit a temperature dependent phase transition, which can be classified as either an upper critical solution temperature (UCST) or lower critical solution temperature (LCST). UCST polymers increase in their water-solubility at higher temperatures, which lead to UCST hydrogels transitioning from a gel (solid) to a solution (liquid) as the temperature is increased (similar to the melting point behavior of pure materials). This phenomenon also causes UCST hydrogels to expand (increase their swell ratio) as temperature increases while they are below their UCST.[46] However, polymers with LCSTs display an inverse (or negative) temperature-dependence, where their water-solubility decreases at higher temperatures. LCST hydrogels transition from a liquid solution to a solid gel as the temperature is increased, and they also shrink (decrease their swell ratio) as the temperature increases while they are above their LCST.[46]
Applications can dictate for diverse thermal responses. For example, in the biomedical field, LCST hydrogels are being investigated as drug delivery systems due to being injectable (liquid) at room temp and then solidifying into a rigid gel upon exposure to the higher temperatures of the human body.[46] There are many other stimuli that hydrogels can be responsive to, including: pH, glucose, electrical signals, light, pressure, ions, antigens, and more.[46]
Additives
The mechanical properties of hydrogels can be fine-tuned in many ways beginning with attention to their hydrophobic properties.[46][47] Another method of modifying the strength or elasticity of hydrogels is to graft or surface coat them onto a stronger/stiffer support, or by making superporous hydrogel (SPH) composites, in which a cross-linkable matrix swelling additive is added.[7] Other additives, such as nanoparticles and microparticles, have been shown to significantly modify the stiffness and gelation temperature of certain hydrogels used in biomedical applications.[48][49][50]
Processing techniques
While a hydrogel's mechanical properties can be tuned and modified through crosslink concentration and additives, these properties can also be enhanced or optimized for various applications through specific processing techniques. These techniques include electro-spinning, 3D/4D printing, self-assembly, and freeze-casting. One unique processing technique is through the formation of multi-layered hydrogels to create a spatially-varying matrix composition and by extension, mechanical properties. This can be done by polymerizing the hydrogel matrixes in a layer by layer fashion via UV polymerization. This technique can be useful in creating hydrogels that mimic articular cartilage, enabling a material with three separate zones of distinct mechanical properties.[51]
Another emerging technique to optimize hydrogel mechanical properties is by taking advantage of the Hofmeister series. Due to this phenomenon, through the addition of salt solution, the polymer chains of a hydrogel aggregate and crystallize, which increases the toughness of the hydrogel. This method, called "salting out", has been applied to poly(vinyl alcohol) hydrogels by adding a sodium sulfate salt solution.[52] Some of these processing techniques can be used synergistically with each other to yield optimal mechanical properties. Directional freezing or freeze-casting is another method in which a directional temperature gradient is applied to the hydrogel is another way to form materials with anisotropic mechanical properties. Utilizing both the freeze-casting and salting-out processing techniques on poly(vinyl alcohol) hydrogels to induce hierarchical morphologies and anisotropic mechanical properties.[53] Directional freezing of the hydrogels helps to align and coalesce the polymer chains, creating anisotropic array honeycomb tube-like structures while salting out the hydrogel yielded out a nano-fibril network on the surface of these honeycomb tube-like structures. While maintaining a water content of over 70%, these hydrogels' toughness values are well above those of water-free polymers such as polydimethylsiloxane (PDMS), Kevlar, and synthetic rubber. The values also surpass the toughness of natural tendon and spider silk.[53]
Applications
Soft contact lenses
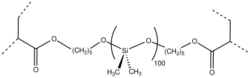
The dominant material for contact lenses are acrylate-siloxane hydrogels. They have replaced hard contact lenses. One of their most attractive properties is oxygen permeability, which is required since the cornea lacks vasculature.
Research
- Coatings for gas evolution reaction electrodes for efficient bubble detachment [55][56][57]
- Breast implants
- Contact lenses (silicone hydrogels, polyacrylamides, polymacon)
- Water sustainability: Hydrogels have emerged as promising materials platforms for solar-powered water purification,[58] water disinfection,[59] and Atmospheric water generator.[60]
- Disposable diapers where they absorb urine, or in sanitary napkins[25]
- Dressings for healing of burn or other hard-to-heal wounds. Wound gels are excellent for helping to create or maintain a moist environment.
- EEG and ECG medical electrodes using hydrogels composed of cross-linked polymers (polyethylene oxide, polyAMPS and polyvinylpyrrolidone)
- Encapsulation of quantum dots
- Environmentally sensitive hydrogels (also known as 'smart gels' or 'intelligent gels'). These hydrogels have the ability to sense changes of pH, temperature, or the concentration of metabolite and release their load as result of such a change.[61][62][63]
- Fibers
- Glue
- Granules for holding soil moisture in arid areas
- Air bubble-repellent (superaerophobicity). Can improve the performance and stability of electrodes for water electrolysis.[64]
- Culturing cells: Hydrogel-coated wells have been used for cell culture.[65]
- Biosensors: Hydrogels that are responsive to specific molecules,[66] such as glucose or antigens, can be used as biosensors, as well as in DDS.[67]
- Cell carrier: Injectable hydrogels can be used to carry drugs or cells for applications in tissue regeneration or 3D bioprinting.[68][69][70] Hydrogels with reversible chemistry are required to allow for fluidization during injection/printing followed by self-healing of the original hydrogel structure.[71]
- Investigate cell biomechanical functions combined with holotomography microscopy
- Provide absorption, desloughing and debriding of necrotic and fibrotic tissue
- Tissue engineering scaffolds. When used as scaffolds, hydrogels may contain human cells to repair tissue. They mimic 3D microenvironment of cells.[72] Materials include agarose, methylcellulose, hyaluronan, elastin-like polypeptides, and other naturally derived polymers.
- Sustained-release drug delivery systems. Ionic strength, pH and temperature can be used as a triggering factor to control the release of the drug.[73]
- Window coating/replacement: Hydrogels are under consideration for reducing infrared light absorption by 75%.[74] Another approach reduced interior temperature using a temperature-responsive hydrogel.[75]
- Thermodynamic electricity generation: When combined with ions allows for heat dissipation for electronic devices and batteries and converting the heat exchange to an electrical charge.[76]
- Water gel explosives
- Controlled release of agrochemicals (pesticides and fertilizer)
- Talin Shock Absorbing Materials - protein-based hydrogels that can absorb supersonic impacts[77]
Biomaterials
Implanted or injected hydrogels have the potential to support tissue regeneration by mechanical tissue support, localized drug or cell delivery,[2] local cell recruitement or immunomodulation, or encapsulation of nanoparticles for local photothermal or brachytherapy.[71] Polymeric drug delivery systems have overcome challenge due to their biodegradability, biocompatibility, and anti-toxicity.[78][79] Materials such as collagen, chitosan, cellulose, and poly (lactic-co-glycolic acid) have been implemented extensively for drug delivery to organs such as eye,[80] nose, kidneys,[81] lungs,[82] intestines,[83] skin[84] and brain.[2] Future work is focused on reducing toxicity, improving biocompatibility, expanding assembly techniques[85]
Hydrogels have been considered as vehicles for drug delivery.[86][68][69][70] They can also be made to mimic animal mucosal tissues to be used for testing mucoadhesive properties.[87][88] They have been examined for use as reservoirs in topical drug delivery; particularly ionic drugs, delivered by iontophoresis.
References
- ↑ Wichterle, O.; Lím, D. (1960-01-01). "Hydrophilic Gels for Biological Use". Nature 185 (4706): 117–118. doi:10.1038/185117a0. ISSN 0028-0836. Bibcode: 1960Natur.185..117W. https://ui.adsabs.harvard.edu/abs/1960Natur.185..117W.
- ↑ 2.0 2.1 2.2 2.3 2.4 2.5 Ghosh, Shampa; Ghosh, Soumya; Sharma, Hitaishi; Bhaskar, Rakesh; Han, Sung Soo; Sinha, Jitendra Kumar (2024-01-01). "Harnessing the power of biological macromolecules in hydrogels for controlled drug release in the central nervous system: A review". International Journal of Biological Macromolecules 254: 127708. doi:10.1016/j.ijbiomac.2023.127708. ISSN 0141-8130. https://www.sciencedirect.com/science/article/pii/S0141813023046068.
- ↑ Shrivastava, Priya; Vishwakarma, Nikhar; Gautam, Laxmikant; Vyas, Suresh P. (2023), "Magnetically responsive polymeric gels and elastomeric system(s) for drug delivery", Smart Polymeric Nano-Constructs in Drug Delivery (Elsevier): pp. 129–150, doi:10.1016/b978-0-323-91248-8.00012-x, ISBN 978-0-323-91248-8, http://dx.doi.org/10.1016/b978-0-323-91248-8.00012-x, retrieved 2023-01-16
- ↑ Fundamental Biomaterials: Polymers. 2018. doi:10.1016/c2016-0-03544-1. ISBN 9780081021941. http://dx.doi.org/10.1016/c2016-0-03544-1.
- ↑ Polymer Science: A Comprehensive Reference. Elsevier. 2012. doi:10.1016/c2009-1-28406-1. ISBN 978-0-08-087862-1. http://dx.doi.org/10.1016/c2009-1-28406-1.
- ↑ 6.0 6.1 "Hydrogels". Kirk-Othmer Encyclopedia of Chemical Technology. 2012. pp. 1–20. doi:10.1002/0471238961.0825041807211620.a01.pub2. ISBN 978-0471238966.
- ↑ 7.0 7.1 "Hydrogel: Preparation, characterization, and applications: A review". Journal of Advanced Research 6 (2): 105–121. March 2015. doi:10.1016/j.jare.2013.07.006. PMID 25750745.
- ↑ "Der Hydrogel und das kristallinische Hydrat des Kupferoxydes". Zeitschrift für Chemie und Industrie der Kolloide 1 (7): 213–214. 1907. doi:10.1007/BF01830147.
- ↑ Nikolić, Ljubiša B.; Zdravković, Aleksandar S.; Nikolić, Vesna D.; Ilić-Stojanović, Snežana S. (2018), Mondal, Md. Ibrahim H., ed., "Synthetic Hydrogels and Their Impact on Health and Environment" (in en), Cellulose-Based Superabsorbent Hydrogels (Cham: Springer International Publishing): pp. 1–29, doi:10.1007/978-3-319-76573-0_61-1, ISBN 978-3-319-76573-0, https://doi.org/10.1007/978-3-319-76573-0_61-1, retrieved 2023-01-17
- ↑ Summonte, S; Racaniello, GF; Lopedota, A; Denora, N; Bernkop-Schnürch, A (2021). "Thiolated polymeric hydrogels for biomedical application: Cross-linking mechanisms". Journal of Controlled Release 330: 470–482. doi:10.1016/j.jconrel.2020.12.037. PMID 33359581.
- ↑ Federer, C; Kurpiers, M; Bernkop-Schnürch, A (2021). "Thiolated Chitosans: A Multi-talented Class of Polymers for Various Applications". Biomacromolecules 22 (1): 24–56. doi:10.1021/acs.biomac.0c00663. PMID 32567846.
- ↑ Leichner, C; Jelkmann, M; Bernkop-Schnürch, A (2019). "Thiolated polymers: Bioinspired polymers utilizing one of the most important bridging structures in nature". Advanced Drug Delivery Reviews 151-152: 191–221. doi:10.1016/j.addr.2019.04.007. PMID 31028759.
- ↑ 13.0 13.1 Rosales, Adrianne M.; Anseth, Kristi S. (2016-02-02). "The design of reversible hydrogels to capture extracellular matrix dynamics" (in en). Nature Reviews Materials 1 (2): 15012. doi:10.1038/natrevmats.2015.12. ISSN 2058-8437. PMID 29214058. Bibcode: 2016NatRM...115012R.
- ↑ Jeong, Byeongmoon; Kim, Sung Wan; Bae, You Han (2002-01-17). "Thermosensitive sol-gel reversible hydrogels". Advanced Drug Delivery Reviews 54 (1): 37–51. doi:10.1016/s0169-409x(01)00242-3. ISSN 0169-409X. PMID 11755705. https://pubmed.ncbi.nlm.nih.gov/11755705/.
- ↑ Yan, Yonggan; Xu, Shulei; Liu, Huanxi; Cui, Xin; Shao, Jinlong; Yao, Peng; Huang, Jun; Qiu, Xiaoyong et al. (2020-05-20). "A multi-functional reversible hydrogel adhesive" (in en). Colloids and Surfaces A: Physicochemical and Engineering Aspects 593: 124622. doi:10.1016/j.colsurfa.2020.124622. ISSN 0927-7757. https://www.sciencedirect.com/science/article/pii/S0927775720302156.
- ↑ Monteiro, O. A.; Airoldi, C. (November 1999). "Some studies of crosslinking chitosan-glutaraldehyde interaction in a homogeneous system". International Journal of Biological Macromolecules 26 (2–3): 119–128. doi:10.1016/s0141-8130(99)00068-9. ISSN 0141-8130. PMID 10517518. https://pubmed.ncbi.nlm.nih.gov/10517518/.
- ↑ Zhang, Zhen; He, Chaoliang; Chen, Xuesi (2018-09-27). "Hydrogels based on pH-responsive reversible carbon–nitrogen double-bond linkages for biomedical applications" (in en). Materials Chemistry Frontiers 2 (10): 1765–1778. doi:10.1039/C8QM00317C. ISSN 2052-1537. https://pubs.rsc.org/en/content/articlelanding/2018/qm/c8qm00317c.
- ↑ "Designing degradable hydrogels for orthogonal control of cell microenvironments". Chemical Society Reviews 42 (17): 7335–7372. September 2013. doi:10.1039/C3CS60040H. PMID 23609001.
- ↑ Jeong, Kwang-Hun; Park, Duckshin; Lee, Young-Chul (July 2017). "Polymer-based hydrogel scaffolds for skin tissue engineering applications: a mini-review" (in en). Journal of Polymer Research 24 (7): 112. doi:10.1007/s10965-017-1278-4. ISSN 1022-9760. http://link.springer.com/10.1007/s10965-017-1278-4.
- ↑ Gdansk University of Technology, Chemical Faculty, Polymer Technology Department, 80-233 Gdansk, ul Narutowicza 11/12; Gibas, Iwona; Janik, Helena (2010-12-15). "Review: Synthetic Polymer Hydrogels for Biomedical Applications". Chemistry & Chemical Technology 4 (4): 297–304. doi:10.23939/chcht04.04.297. http://science2016.lp.edu.ua/chcht/review-synthetic-polymer-hydrogels-biomedical-applications.
- ↑ "Peptide and Protein Hydrogels.". Polymeric and self assembled hydrogels: from fundamental understanding to applications.. Monographs in supramolecular chemistry.. 11. Cambridge, UK: Royal Society of Chemistry. 2013. pp. 93–124. ISBN 978-1-84973-561-2. https://authors.library.caltech.edu/38546/.
- ↑ "Functionalized α-Helical Peptide Hydrogels for Neural Tissue Engineering". ACS Biomaterials Science & Engineering 1 (6): 431–439. June 2015. doi:10.1021/acsbiomaterials.5b00051. PMID 26240838.
- ↑ "Rational design and application of responsive alpha-helical peptide hydrogels". Nature Materials 8 (7): 596–600. July 2009. doi:10.1038/nmat2479. PMID 19543314. Bibcode: 2009NatMa...8..596B.
- ↑ 24.0 24.1 "Recent advances in photo-crosslinkable hydrogels for biomedical applications". BioTechniques 66 (1): 40–53. January 2019. doi:10.2144/btn-2018-0083. PMID 30730212.
- ↑ 25.0 25.1 "Biomedical applications of hydrogels: A review of patents and commercial products". European Polymer Journal 65: 252–267. 2015. doi:10.1016/j.eurpolymj.2014.11.024.
- ↑ Adelnia, Hossein; Ensandoost, Reza; Shebbrin Moonshi, Shehzahdi; Gavgani, Jaber Nasrollah; Vasafi, Emad Izadi; Ta, Hang Thu (2022-02-05). "Freeze/thawed polyvinyl alcohol hydrogels: Present, past and future" (in en). European Polymer Journal 164: 110974. doi:10.1016/j.eurpolymj.2021.110974. ISSN 0014-3057. https://www.sciencedirect.com/science/article/pii/S0014305721007084.
- ↑ Augst, Alexander D.; Kong, Hyun Joon; Mooney, David J. (2006-08-07). "Alginate Hydrogels as Biomaterials" (in en). Macromolecular Bioscience 6 (8): 623–633. doi:10.1002/mabi.200600069. ISSN 1616-5187. PMID 16881042. https://onlinelibrary.wiley.com/doi/10.1002/mabi.200600069.
- ↑ Jaipan, Panupong; Nguyen, Alexander; Narayan, Roger J. (2017-09-01). "Gelatin-based hydrogels for biomedical applications" (in en). MRS Communications 7 (3): 416–426. doi:10.1557/mrc.2017.92. ISSN 2159-6867. Bibcode: 2017MRSCo...7..416J.
- ↑ "Self-assembly of short peptides to form hydrogels: design of building blocks, physical properties and technological applications". Acta Biomaterialia 10 (4): 1671–1682. April 2014. doi:10.1016/j.actbio.2013.08.013. PMID 23958781.
- ↑ "Nanostructured Hydrogels for Three-Dimensional Cell Culture Through Self-Assembly of Fluorenylmethoxycarbonyl–Dipeptides" (in en). Advanced Materials 18 (5): 611–614. 2006-03-03. doi:10.1002/adma.200501522. ISSN 0935-9648. Bibcode: 2006AdM....18..611J. https://onlinelibrary.wiley.com/doi/10.1002/adma.200501522.
- ↑ "Self-assembled Fmoc-peptides as a platform for the formation of nanostructures and hydrogels". Biomacromolecules 10 (9): 2646–2651. September 2009. doi:10.1021/bm900584m. PMID 19705843.
- ↑ "The delicate balance between gelation and crystallisation: structural and computational investigations" (in en). Soft Matter 6 (17): 4144. 2010. doi:10.1039/c0sm00409j. ISSN 1744-683X. Bibcode: 2010SMat....6.4144A. http://xlink.rsc.org/?DOI=c0sm00409j.
- ↑ 33.0 33.1 "Self-assembly mechanism for a naphthalene-dipeptide leading to hydrogelation". Langmuir 26 (7): 5232–5242. April 2010. doi:10.1021/la903694a. PMID 19921840.
- ↑ 34.0 34.1 "Relationship between molecular structure, gelation behaviour and gel properties of Fmoc-dipeptides". Soft Matter 6 (9): 1971. 2010. doi:10.1039/b921863g. ISSN 1744-683X. Bibcode: 2010SMat....6.1971A. http://xlink.rsc.org/?DOI=b921863g.
- ↑ "Chemically programmed self-sorting of gelator networks". Nature Communications 4 (1): 1480. June 2013. doi:10.1038/ncomms2499. PMID 23403581. Bibcode: 2013NatCo...4.1480M.
- ↑ "Unzipping the role of chirality in nanoscale self-assembly of tripeptide hydrogels". Nanoscale 4 (21): 6752–6760. November 2012. doi:10.1039/c2nr32006a. PMID 22955637. Bibcode: 2012Nanos...4.6752M.
- ↑ "Exploiting CH-π interactions in supramolecular hydrogels of aromatic carbohydrate amphiphiles" (in en). Chemical Science 2 (7): 1349. 2011. doi:10.1039/c0sc00621a. ISSN 2041-6520. http://xlink.rsc.org/?DOI=c0sc00621a.
- ↑ "Aromatic-aromatic interactions induce the self-assembly of pentapeptidic derivatives in water to form nanofibers and supramolecular hydrogels". Journal of the American Chemical Society 132 (8): 2719–2728. March 2010. doi:10.1021/ja9088764. PMID 20131781.
- ↑ "A low-energy-consumption electroactive valveless hydrogel micropump for long-term biomedical applications". Lab on a Chip 11 (17): 2910–2915. September 2011. doi:10.1039/C1LC20288J. PMID 21761057.
- ↑ 40.0 40.1 40.2 40.3 40.4 40.5 40.6 40.7 40.8 "Mechanical characterisation of hydrogel materials" (in en). International Materials Reviews 59 (1): 44–59. January 2014. doi:10.1179/1743280413Y.0000000022. ISSN 0950-6608. Bibcode: 2014IMRv...59...44O.
- ↑ (in en) Stem Cells and Biomaterials for Regenerative Medicine. Academic Press. 2018-11-07. ISBN 978-0-12-812278-5. https://books.google.com/books?id=B293DwAAQBAJ&q=Matching%20the%20modulus%20with%20the%20surrounding%20tissue&pg=PA95.
- ↑ "Strain rate viscoelastic analysis of soft and highly hydrated biomaterials". Journal of Biomedical Materials Research. Part A 102 (10): 3352–3360. October 2014. doi:10.1002/jbm.a.34914. PMID 23946054.
- ↑ 43.0 43.1 "Mechanical properties of hydrogels and their experimental determination". Biomaterials 17 (17): 1647–1657. September 1996. doi:10.1016/0142-9612(96)87644-7. PMID 8866026.
- ↑ ""Engineering viscoelasticity"". Massachusetts Institute of Technology. http://web.mit.edu/course/3/3.11/www/modules/visco.pdf.
- ↑ 45.0 45.1 "Poroelasticity of cellulose hydrogel". Journal of the Taiwan Institute of Chemical Engineers 92: 118–122. November 2018. doi:10.1016/j.jtice.2018.02.017.
- ↑ 46.0 46.1 46.2 46.3 46.4 46.5 "Environment-sensitive hydrogels for drug delivery". Advanced Drug Delivery Reviews 53 (3): 321–339. December 2001. doi:10.1016/S0169-409X(01)00203-4. PMID 11744175.
- ↑ "Effect of crosslinker length on the elastic and compression modulus of poly(acrylamide) nanocomposite hydrogels" (in en). Journal of Physics: Conference Series 790 (1): 012037. January 2017. doi:10.1088/1742-6596/790/1/012037. ISSN 1742-6588. Bibcode: 2017JPhCS.790a2037Z.
- ↑ "Injectable Hydrogels Based on Pluronic/Water Systems Filled with Alginate Microparticles for Biomedical Applications". Materials 12 (7): 1083. April 2019. doi:10.3390/ma12071083. PMID 30986948. Bibcode: 2019Mate...12.1083C.
- ↑ "Nanoparticle solutions as adhesives for gels and biological tissues". Nature 505 (7483): 382–385. January 2014. doi:10.1038/nature12806. PMID 24336207. Bibcode: 2014Natur.505..382R.
- ↑ "Exploring the Role of Nanoparticles in Enhancing Mechanical Properties of Hydrogel Nanocomposites". Nanomaterials 8 (11): 882. October 2018. doi:10.3390/nano8110882. PMID 30380606.
- ↑ "Engineering articular cartilage with spatially-varying matrix composition and mechanical properties from a single stem cell population using a multi-layered hydrogel". Biomaterials 32 (29): 6946–6952. October 2011. doi:10.1016/j.biomaterials.2011.06.014. PMID 21723599.
- ↑ "4D Printable Tough and Thermoresponsive Hydrogels". ACS Applied Materials & Interfaces 13 (11): 12689–12697. March 2021. doi:10.1021/acsami.0c17532. PMID 33263991.
- ↑ 53.0 53.1 "Strong tough hydrogels via the synergy of freeze-casting and salting out". Nature 590 (7847): 594–599. February 2021. doi:10.1038/s41586-021-03212-z. PMID 33627812. Bibcode: 2021Natur.590..594H.
- ↑ "Contact Lenses". Kirk‐Othmer Encyclopedia of Chemical Technology. John Wiley & Sons, Inc. 2000. doi:10.1002/0471238961. ISBN 9780471484943.
- ↑ Jeon, Dasom; Park, Jinwoo; Shin, Changhwan; Kim, Hyunwoo; Jang, Ji-Wook; Lee, Dong Woog; Ryu, Jungki (2020-04-10). "Superaerophobic hydrogels for enhanced electrochemical and photoelectrochemical hydrogen production" (in en). Science Advances 6 (15): eaaz3944. doi:10.1126/sciadv.aaz3944. ISSN 2375-2548. PMID 32300656. Bibcode: 2020SciA....6.3944J.
- ↑ Bae, Misol; Kang, Yunseok; Lee, Dong Woog; Jeon, Dasom; Ryu, Jungki (August 2022). "Superaerophobic Polyethyleneimine Hydrogels for Improving Electrochemical Hydrogen Production by Promoting Bubble Detachment" (in en). Advanced Energy Materials 12 (29): 2201452. doi:10.1002/aenm.202201452. ISSN 1614-6832.
- ↑ Park, Jinwoo; Jeon, Dasom; Kang, Yunseok; Ryu, Jungki; Lee, Dong Woog (2023-01-24). "Nanofibrillar hydrogels outperform Pt/C for hydrogen evolution reactions under high-current conditions" (in en). Journal of Materials Chemistry A 11 (4): 1658–1665. doi:10.1039/D2TA08775H. ISSN 2050-7496. https://pubs.rsc.org/en/content/articlelanding/2023/ta/d2ta08775h.
- ↑ Youhong Guo; H. Lu; F. Zhao; X. Zhou; W. Shi; Guihua Yu (2020). "Biomass-Derived Hybrid Hydrogel Evaporators for Cost-Effective Solar Water Purification". Advanced Materials 32 (11): 1907061. doi:10.1002/adma.201907061. PMID 32022974. Bibcode: 2020AdM....3207061G.
- ↑ Youhong Guo; C. M. Dundas; X. Zhou; K. P. Johnston; Guihua Yu (2021). "Molecular Engineering of Hydrogels for Rapid Water Disinfection and Sustainable Solar Vapor Generation". Advanced Materials 33 (35): 2102994. doi:10.1002/adma.202102994. PMID 34292641. Bibcode: 2021AdM....3302994G.
- ↑ Youhong Guo; W. Guan; C. Lei; H. Lu; W. Shi; Guihua Yu (2022). "Scalable super hygroscopic polymer films for sustainable moisture harvesting in arid environments". Nature Communications 13 (1): 2761. doi:10.1038/s41467-022-30505-2. PMID 35589809. Bibcode: 2022NatCo..13.2761G.
- ↑ "On-demand drug delivery from local depots". Journal of Controlled Release 219: 8–17. December 2015. doi:10.1016/j.jconrel.2015.09.011. PMID 26374941.
- ↑ "Bioinspired mechanically active adhesive dressings to accelerate wound closure". Science Advances 5 (7): eaaw3963. July 2019. doi:10.1126/sciadv.aaw3963. PMID 31355332. Bibcode: 2019SciA....5.3963B.
- ↑ "Smart hydrogels for advanced drug delivery systems". International Journal of Molecular Sciences 23 (7): 3665. 2022. doi:10.3390/ijms23073665. PMID 35409025.
- ↑ "Superaerophobic hydrogels for enhanced electrochemical and photoelectrochemical hydrogen production". Science Advances 6 (15): eaaz3944. April 2020. doi:10.1126/sciadv.aaz3944. PMID 32300656. Bibcode: 2020SciA....6.3944J.
- ↑ "Tissue cells feel and respond to the stiffness of their substrate". Science 310 (5751): 1139–1143. November 2005. doi:10.1126/science.1116995. PMID 16293750. Bibcode: 2005Sci...310.1139D.
- ↑ Chemoresponsive Materials. Cambridge: Royal Society of Chemistry. 2015. ISBN 978-1-78262-242-0. https://pubs.rsc.org/en/content/ebook/.
- ↑ "Holographic sensors: three-dimensional analyte-sensitive nanostructures and their applications". Chemical Reviews 114 (20): 10654–10696. October 2014. doi:10.1021/cr500116a. PMID 25211200.
- ↑ 68.0 68.1 "Injectable hydrogels delivering therapeutic agents for disease treatment and tissue engineering". Biomaterials Research 22 (1): 27. December 2018. doi:10.1186/s40824-018-0138-6. PMID 30275970.
- ↑ 69.0 69.1 "Injectable hydrogels for cartilage and bone tissue engineering". Bone Research 5 (1): 17014. December 2017. doi:10.1038/boneres.2017.14. PMID 28584674.
- ↑ 70.0 70.1 "Injectable Shape-Holding Collagen Hydrogel for Cell Encapsulation and Delivery Cross-linked Using Thiol-Michael Addition Click Reaction". Biomacromolecules 20 (9): 3475–3484. September 2019. doi:10.1021/acs.biomac.9b00769. PMID 31408340.
- ↑ 71.0 71.1 Bertsch, Pascal; Diba, Mani; Mooney, David J.; Leeuwenburgh, Sander C. G. (25 January 2023). "Self-Healing Injectable Hydrogels for Tissue Regeneration". Chemical Reviews 123 (2): 834–873. doi:10.1021/acs.chemrev.2c00179. PMID 35930422.
- ↑ "A biodegradable thermosensitive hydrogel with tuneable properties for mimicking three-dimensional microenvironments of stem cells". RSC Adv. 4 (109): 63951–63961. 2014. doi:10.1039/C4RA12215A. ISSN 2046-2069. Bibcode: 2014RSCAd...463951M.
- ↑ "Biomacromolecules in microgels — Opportunities and challenges for drug delivery". Current Opinion in Colloid & Interface Science 15 (6): 435–444. 2010-12-01. doi:10.1016/j.cocis.2010.05.016. ISSN 1359-0294.
- ↑ Irving, Michael (2022-08-31). "Hydrogel glass windows let in more light and less heat" (in en-US). https://newatlas.com/materials/hydrogel-glass-windows-more-light-less-heat/.
- ↑ Miller, Brittney J. (8 June 2022). "How smart windows save energy". Knowable Magazine. doi:10.1146/knowable-060822-3. https://knowablemagazine.org/article/technology/2022/how-smart-windows-save-energy. Retrieved 15 July 2022.
- ↑ "A new way to cool down electronic devices, recover waste heat". April 22, 2020. https://phys.org/news/2020-04-cool-electronic-devices-recover.html.
- ↑ Lavars, Nick (2022-12-15). "New protein-based armor material can withstand supersonic impacts" (in en-US). https://newatlas.com/materials/protein-based-armor-material-withstand-supersonic-impacts/.
- ↑ "Physical hydrogels with self-assembled nanostructures as drug delivery systems". Expert Opinion on Drug Delivery 8 (9): 1141–1159. September 2011. doi:10.1517/17425247.2011.588205. PMID 21619469.
- ↑ "Defining and designing polymers and hydrogels for neural tissue engineering". Neuroscience Research 72 (3): 199–213. March 2012. doi:10.1016/j.neures.2011.12.005. PMID 22192467.
- ↑ "Ultrathin chitosan-poly(ethylene glycol) hydrogel films for corneal tissue engineering". Acta Biomaterialia 9 (5): 6594–6605. May 2013. doi:10.1016/j.actbio.2013.01.020. PMID 23376126.
- ↑ "The use of chitosan based hydrogel for enhancing the therapeutic benefits of adipose-derived MSCs for acute kidney injury". Biomaterials 33 (14): 3673–3681. May 2012. doi:10.1016/j.biomaterials.2012.01.061. PMID 22361096.
- ↑ "Sealing effect of rapidly curable gelatin-poly (L-glutamic acid) hydrogel glue on lung air leak". The Annals of Thoracic Surgery 67 (4): 922–926. April 1999. doi:10.1016/S0003-4975(99)00153-8. PMID 10320229.
- ↑ "Alginate encapsulated bioadhesive chitosan microspheres for intestinal drug delivery". Journal of Biomaterials Applications 13 (4): 290–296. April 1999. doi:10.1177/088532829901300402. PMID 10340211.
- ↑ "Chitosan-Based Biomaterials for Tissue Repair and Regeneration" (in en), Chitosan for Biomaterials II, Advances in Polymer Science (Springer Berlin Heidelberg) 244: pp. 81–127, 2011, doi:10.1007/12_2011_118, ISBN 978-3-642-24061-4
- ↑ "Hydrogels with self-assembling ordered structures and their functions" (in en). NPG Asia Materials 3 (6): 57–64. June 2011. doi:10.1038/asiamat.2010.200. ISSN 1884-4057.
- ↑ "Three-dimensional porous biodegradable polymeric scaffolds fabricated with biodegradable hydrogel porogens". Tissue Engineering. Part C, Methods 15 (4): 583–594. December 2009. doi:10.1089/ten.TEC.2008.0642. PMID 19216632.
- ↑ "Novel glycopolymer hydrogels as mucosa-mimetic materials to reduce animal testing". Chemical Communications 51 (77): 14447–14450. October 2015. doi:10.1039/C5CC02428E. PMID 26221632.
- ↑ "Mucoadhesion and mucosa-mimetic materials--A mini-review". International Journal of Pharmaceutics 495 (2): 991–998. November 2015. doi:10.1016/j.ijpharm.2015.09.064. PMID 26440734.
Further reading
- "The Preparation and Simple Analysis of a Clay Nanoparticle Composite Hydrogel". Journal of Chemical Education 94 (11): 1772–1779. 2017. doi:10.1021/acs.jchemed.6b00389. ISSN 0021-9584. Bibcode: 2017JChEd..94.1772W.
![]() | Original source: https://en.wikipedia.org/wiki/Hydrogel.
Read more |