Physics:Nanocluster
Nanoclusters are atomically precise, crystalline materials most often existing on the 0-2 nanometer scale.[1] [2][3] They are often considered kinetically stable intermediates that form during the synthesis of comparatively larger materials such as semiconductor and metallic nanocrystals. The majority of research conducted to study nanoclusters has focused on characterizing their crystal structures and understanding their role in the nucleation and growth mechanisms of larger materials.[4][5] These nanoclusters can be composed either of a single or of multiple elements, and exhibit interesting electronic, optical, and chemical properties compared to their larger counterparts.[3][2][6][7]
Materials can be categorized into three different regimes, namely bulk, nanoparticles and nanoclusters. Bulk metals are electrical conductors and good optical reflectors and metal nanoparticles display intense colors due to surface plasmon resonance.[6][7] However, when the size of metal nanoclusters is further reduced to form a nanocluster, the band structure becomes discontinuous and breaks down into discrete energy levels, somewhat similar to the energy levels of molecules.[6][7][8][9][10] This gives nanoclusters similar qualities as a singular molecule[11] and does not exhibit plasmonic behavior; nanoclusters are known as the bridging link between atoms and nanoparticles.[12][6][7][8][9][10][13][14][15][16][17] Nanoclusters may also be referred to as molecular nanoparticles.[18]
Atom clusters
In chemistry, an atom cluster (or simply cluster) is an ensemble of bound atoms or molecules that is intermediate in size between a simple molecule and a nanoparticle; that is, up to a few nanometers (nm) in diameter. The term microcluster may be used for ensembles with up to couple dozen atoms.
Clusters with a definite number and type of atoms in a specific arrangement are often considered a specific chemical compound and are studied as such. For example, fullerene is a cluster of 60 carbon atoms arranged as the vertices of a truncated icosahedron, and decaborane is a cluster of 10 boron atoms forming an incomplete icosahedron, surrounded by 14 hydrogen atoms.
The term is most commonly used for ensembles consisting of several atoms of the same element, or of a few different elements, bonded in a three-dimensional arrangement. Transition metals and main group elements form especially robust clusters.[19] Indeed, in some contexts, the term may refer specifically to a metal cluster, whose core atoms are metals and contains at least one metallic bond.[20] In this case, the qualifier poly specifies a cluster with more than one metal atom, and heteronuclear specifies a cluster with at least two different metal elements. Naked metal clusters have only metal atoms, as opposed to clusters with outer shell of other elements. The latter may be functional groups such as cyanide or methyl, covalently bonded to the core atoms; or many be ligands attached by coordination bonds, such as carbon monoxide, halides, isocyanides, alkenes, and hydrides.
However, the term is also used for ensembles that contain no metals (such as the boranes and carboranes) and whose core atoms are held together by covalent or ionic bonds. It is also used for ensembles of atoms or molecules held together by Van der Waals or hydrogen bonds, as in water clusters.
Clusters may play an important role in phase transitions such as precipitation from solutions, condensation and evaporation of liquids and solids, freezing and melting, and adsorption to other materials.[citation needed]
History
Atom cluster compounds, including metal clusters, have been unwittingly used by humans since antiquity. The oldest artificially produced metal cluster may be calomel Hg2Cl2, which was known in India already in the 12th century.
The elucidation of the structure of cluster compounds only became possible in the 20th century. For instance, the existence of a mercury to mercury bond in calomel was established in the early 1900s. These advances were made possible by the development of reliable structural analysis tools, such as single-crystal X-ray diffraction.
The first set of experiments to form nanoclusters can be traced back to 1950s and 1960s.[22][13] During this period, nanoclusters were produced from intense molecular beams at low temperature by supersonic expansion. The development of laser vaporization technique made it possible to create nanoclusters of a clear majority of the elements in the periodic table.
The term "cluster" was used by F.A. Cotton in the early 1960s to refer specifically to compounds containing metal–metal bonds.
Carbon clusters were first detected by Eric A. Rohlfing, Donald M. Cox, and Andrew Kaldor in 1984, in experiments where graphite was vaporized by laser and the vapor was quenched by a helium atmosphere. Analysis of the condensed products with a mass spectrometer revealed a preponderance of molecules with certain "magic numbers".[23] In 1985 their work was repeated by Harold Kroto, James R. Heath, Sean O'Brien, Robert Curl, and Richard Smalley, who proposed the truncated icosahedron structure for the prominent C60 molecule, and proposed the name buckminsterfullerene for it.[24]
Since 1980s, there has been tremendous work on nanoclusters of semiconductor elements, compound clusters and transition metal nanoclusters.[13]
Size and number of atoms in metal nanoclusters
According to the Japanese mathematical physicist Ryogo Kubo, the spacing of energy levels can be predicted by
[math]\displaystyle{ \delta=\frac{E_{\rm F}}{N} }[/math]
where EF is Fermi energy and N is the number of atoms. For quantum confinement 𝛿 can be estimated to be equal to the thermal energy (δ = kT), where k is the Boltzmann constant and T is temperature.[25][26]
Structure and stability

The physical and chemical properties of atom clusters are very different from those of bulk solid with the same composition. The difference is due to the fact that a large fraction of their component atoms is found at their surface. For cluster cores with fewer than a couple dozen component atoms or molecules, the stable configurations usually have most or all atoms adjacent to the core's surface, and thus only partially bound to other core elements.
A gradual transition occurs between the properties of the molecular species and those of the corresponding bulk mix with increasing number N of atoms in the core, since the fraction of atoms adjacent to its surface will scale approximately as N−1/3. If N is 105, when the cluster can be considered a nanoparticle, only about 10% of the atoms in the core will be exposed at its surface. That is still significant percentage, which is part of the reason why the properties of nanoparticles are still significantly different from those of the bulk substance.
Transition metal clusters are frequently composed of refractory metal atoms. In general metal centers with extended d-orbitals form stable clusters because of favorable overlap of valence orbitals. Thus, metals with a low oxidation state for the later metals and mid-oxidation states for the early metals tend to form stable clusters. Polynuclear metal carbonyls are generally found in late transition metals with low formal oxidation states. The polyhedral skeletal electron pair theory or Wade's electron counting rules predict trends in the stability and structures of many metal clusters. Jemmis mno rules have provided additional insight into the relative stability of metal clusters.
Not all the clusters are stable. The stability of nanoclusters depends on the number of atoms in the nanocluster, valence electron counts and encapsulating scaffolds.[28] In the 1990s, Heer and his coworkers used supersonic expansion of an atomic cluster source into a vacuum in the presence of an inert gas and produced atomic cluster beams.[26] Heer's team and Brack et al. discovered that certain masses of formed metal nanoclusters were stable and were like magic clusters.[29] The number of atoms or size of the core of these magic clusters corresponds to the closing of atomic shells. Certain thiolated clusters such as Au25(SR)18, Au38(SR)24, Au102(SR)44 and Au144(SR)60 also showed magic number stability.[8] Häkkinen et al explained this stability with a theory that a nanocluster is stable if the number of valence electrons corresponds to the shell closure of atomic orbitals as (1S2, 1P6, 1D10, 2S2 1F14, 2P6 1G18, 2D10 3S2 1H22.......).[30][31]
Gas-phase clusters and fullerenes
Unstable clusters can also be observed in the gas-phase by means of mass spectrometry, even though they may be thermodynamically unstable and aggregate easily upon condensation. Such naked clusters, i.e. those that are not stabilized by ligands, are often produced by laser induced evaporation - or ablation - of a bulk metal or metal-containing compound. Typically, this approach produces a broad distribution of size distributions. Their electronic structures can be interrogated by techniques such as photoelectron spectroscopy, while infrared multiphoton dissociation spectroscopy is more probing the clusters geometry.[32] Their properties (Reactivity, Ionization potential, HOMO–LUMO-gap) often show a pronounced size dependence. Examples of such clusters are certain aluminium clusters as superatoms and certain gold clusters. Certain metal clusters are considered to exhibit metal aromaticity. In some cases, the results of laser ablation experiments are translated to isolated compounds, and the premier cases are the clusters of carbon called the fullerenes, notably clusters with the formula C60, C70, and C84. The fullerene sphere can be filled with small molecules, forming Endohedral fullerenes.
Synthesis and stabilization
Solid state medium
Molecular beams can be used to create nanocluster beams of virtually any element. They can be synthesized in high vacuum by with molecular beam techniques combined with a mass spectrometer for mass selection, separation and analysis. And finally detected with detectors.[33]
Cluster Sources
Seeded supersonic nozzle Seeded supersonic nozzles are mostly used to create clusters of low-boiling-point metal. In this source method metal is vaporized in a hot oven. The metal vapor is mixed with (seeded in) inert carrier gas. The vapor mixture is ejected into a vacuum chamber via a small hole, producing a supersonic molecular beam. The expansion into vacuum proceeds adiabatically cooling the vapor. The cooled metal vapor becomes supersaturated, condensing in cluster form.
Gas aggregation Gas aggregation is mostly used to synthesize large clusters of nanoparticles. Metal is vaporized and introduced in a flow of cold inert gas, which causes the vapor to become highly supersaturated. Due to the low temperature of the inert gas, cluster production proceeds primarily by successive single-atom addition.
Laser vaporization Laser vaporization source can be used to create clusters of various size and polarity. Pulse laser is used to vaporize the target metal rod and the rod is moved in a spiral so that a fresh area can be evaporated every time. The evaporated metal vapor is cooled by using cold helium gas, which causes the cluster formation.
Pulsed arc cluster ion This is similar to laser vaporization, but an intense electric discharge is used to evaporate the target metal.
Ion sputtering Ion sputtering source produces an intense continuous beam of small singly ionized cluster of metals. Cluster ion beams are produced by bombarding the surface with high energetic inert gas (krypton and xenon) ions. The cluster production process is still not fully understood.
Liquid-metal ion In liquid-metal ion source a needle is wetted with the metal to be investigated. The metal is heated above the melting point and a potential difference is applied. A very high electric field at the tip of the needle causes a spray of small droplets to be emitted from the tip. Initially very hot and often multiply ionized droplets undergo evaporative cooling and fission to smaller clusters.
Mass Analyzer
Wien filter. In Wien filter mass separation is done with crossed homogeneous electric and magnetic fields perpendicular to ionized cluster beam. The net force on a charged cluster with mass M, charge Q, and velocity v vanishes if E = Bv/c. The cluster ions are accelerated by a voltage V to an energy QV. Passing through the filter, clusters with M/Q = 2V/(Ec/B) are un-deflected. The un-deflected cluster ions are selected with appropriately positioned collimators.
Quadrupole mass filter. The quadrupole mass filter operates on the principle that ion trajectories in a two-dimensional quadrupole field are stable if the field has an AC component superimposed on a DC component with appropriate amplitudes and frequencies. It is responsible for filtering sample ions based on their mass-to-charge ratio.
Time of flight mass spectroscopy. Time-of-flight spectroscopy consists of an ion gun, a field-free drift space and an ion cluster source. The neutral clusters are ionized, typically using pulsed laser or an electron beam. The ion gun accelerates the ions that pass through the field-free drift space (flight tube) and ultimately impinge on an ion detector. Usually an oscilloscope records the arrival time of the ions. The mass is calculated from the measured time of flight.
Molecular beam chromatography. In this method, cluster ions produced in a laser vaporized cluster source are mass selected and introduced in a long inert-gas-filled drift tube with an entrance and exit aperture. Since cluster mobility depends upon the collision rate with the inert gas, they are sensitive to the cluster shape and size.
Aqueous medium
In general, metal nanoclusters in an aqueous medium are synthesized in two steps: reduction of metal ions to zero-valent state and stabilization of nanoclusters. Without stabilization, metal nanoclusters would strongly interact with each other and aggregate irreversibly to form larger particles.
Reduction
There are several methods reported to reduce silver ion into zero-valent silver atoms:
- Chemical Reduction. Chemical reductants can reduce silver ions into silver nanoclusters. Some examples of chemical reductants are sodium borohydride (NaBH4) and sodium hypophosphite (NaPO2H2.H2O). For instance, Dickson and his research team have synthesized silver nanoclusters in DNA using sodium borohydride.[15][14]
- Electrochemical Reduction. Silver nanoclusters can also be reduced electrochemically using reductants in the presence of stabilizing agents such as dodecanethiol and tetrabutylammonium.[17]
- Photoreduction. Silver nanoclusters can be produced using ultraviolet light, visible or infrared light. The photoreduction process has several advantages such as avoiding the introduction of impurities, fast synthesis, and controlled reduction. For example Diaz and his co-workers have used visible light to reduce silver ions into nanoclusters in the presence of a PMAA polymer. Kunwar et al produced silver nanoclusters using infrared light.[2][6]
- Other reduction methods. Silver nanoclusters are also formed by reducing silver ions with gamma rays, microwaves, or ultrasound. For example silver nanoclusters formed by gamma reduction technique in aqueous solutions that contain sodium polyacrylate or partly carboxylated polyacrylamide or glutaric acids. By irradiating microwaves Linja Li prepared fluorescent silver nanoclusters in PMAA, which typically possess a red color emission. Similarly Suslick et al. have synthesized silver nanoclusters using high ultrasound in the presence of PMAA polymer.[6][16]
Stabilization
Cryogenic gas molecules are used as scaffolds for nanocluster synthesis in solid state.[9] In aqueous medium there are two common methods for stabilizing nanoclusters: electrostatic (charge, or inorganic) stabilization and steric (organic) stabilization. Electrostatic stabilization occurs by the adsorption of ions to the often-electrophilic metal surface, which creates an electrical double layer. Thus, this Coulomb repulsion force between individual particles will not allow them to flow freely without agglomeration. Whereas on the other hand in steric stabilization,the metal center is surrounded by layers of sterically bulk material. These large adsorbates provide a steric barrier which prevents close contact of the metal particle centers.[6]
Thiols. Thiol-containing small molecules are the most commonly adopted stabilizers in metal nanoparticle synthesis owing to the strong interaction between thiols and gold and silver. Glutathione has been shown to be an excellent stabilizer for synthesizing gold nanoclusters with visible luminescence by reducing Au3+ in the presence of glutathione with sodium borohydride (NaBH4). Also other thiols such as tiopronin, phenylethylthiolate, thiolate α-cyclodextrin and 3-mercaptopropionic acid and bidentate dihydrolipoic acid are other thiolated compounds currently being used in the synthesis of metal nanoclusters. The size as well as the luminescence efficiency of the nanocluster depends sensitively on the thiol-to-metal molar ratio. The higher the ratio, the smaller the nanoclusters. The thiol-stabilized nanoclusters can be produced using strong as well as mild reductants. Thioled metal nanoclusters are mostly produced using the strong reductant sodium borohydride (NaBH4). Gold nanocluster synthesis can also be achieved using a mild reducant tetrakis(hydroxymethyl)phosphonium (THPC). Here a zwitterionic thiolate ligand, D-penicillamine (DPA), is used as the stabilizer. Furthermore, nanoclusters can be produced by etching larger nanoparticles with thiols. Thiols can be used to etch larger nanoparticles stabilized by other capping agents.
![]() |
Look up mercaptoundecanoic acid or microgel in Wiktionary, the free dictionary. |
Dendrimers. Dendrimers are used as templates to synthesize nanoclusters. Gold nanoclusters embedded in poly(amidoamine) dendrimer (PAMAM) have been successfully synthesized. PAMAM is repeatedly branched molecules with different generations. The fluorescence properties of the nanoclusters are sensitively dependent on the types of dendrimers used as template for the synthesis. Metal nanoclusters embedded in different templates show maximum emission at different wavelengths. The change in fluorescence property is mainly due to surface modification by the capping agents. Although gold nanoclusters embedded in PAMAM are blue-emitting the spectrum can be tuned from the ultraviolet to the near-infrared (NIR) region and the relative PAMAM/gold concentration and the dendrimer generation can be varied. The green-emitting gold nanoclusters can be synthesized by adding mercaptoundecanoic acid (MUA) into the prepared small gold nanoparticle solution. The addition of freshly reduced lipoic acid (DHLA) gold nanoclusters (AuNC@DHLA) become red-emitting fluorophores.[6][7]
Polymers. Polymers with abundant carboxylic acid groups were identified as promising templates for synthesizing highly fluorescent, water-soluble silver nanoclusters. Fluorescent silver nanoclusters have been successfully synthesized on poly(methacrylic acid), microgels of poly(N-isopropylacrylamide-acrylic acid-2-hydroxyethyl acrylate) polyglycerol-block-poly(acrylic acid) copolymers polyelectrolyte, poly(methacrylic acid) (PMAA) etc.[10] Gold nanoclusters have been synthesized with polyethylenimine (PEI) and poly(N-vinylpyrrolidone) (PVP) templates. The linear polyacrylates, poly(methacrylic acid), act as an excellent scaffold for the preparation of silver nanoclusters in water solution by photoreduction. Poly(methacrylic acid)-stabilized nanoclusters have an excellent high quantum yield and can be transferred to other scaffolds or solvents and can sense the local environment.[2][6][7][8][9][34][35]
DNA, proteins and peptides. DNA oligonucleotides are good templates for synthesizing metal nanoclusters. Silver ions possess a high affinity to cytosine bases in single-stranded DNA which makes DNA a promising candidate for synthesizing small silver nanoclusters. The number of cytosines in the loop could tune the stability and fluorescence of Ag NCs. Biological macromolecules such as peptides and proteins have also been utilized as templates for synthesizing highly fluorescent metal nanoclusters. Compared with short peptides, large and complicated proteins possess abundant binding sites that can potentially bind and further reduce metal ions, thus offering better scaffolds for template-driven formation of small metal nanoclusters. Also the catalytic function of enzymes can be combined with the fluorescence property of metal nanoclusters in a single cluster to make it possible to construct multi-functional nanoprobes.[6][8][9][7][15]
Inorganic scaffolds. Inorganic materials like glass and zeolite are also used to synthesize the metal nanoclusters. Stabilization is mainly by immobilization of the clusters and thus preventing their tendency to aggregate to form larger nanoparticles. First metal ions doped glasses are prepared and later the metal ion doped glass is activated to form fluorescent nanoclusters by laser irradiation. In zeolites, the pores which are in the Ångström size range can be loaded with metal ions and later activated either by heat treatment, UV light excitation, or two-photon excitation. During the activation, the silver ions combine to form the nanoclusters that can grow only to oligomeric size due to the limited cage dimensions.[6][36]
small molecules. Some small molecules are also used as stabilizers or reductants for the synthesis of CuNCs. These small molecules are usually thiols or carboxyl groups, which exhibit good reducibility for metal salts and affinity for metal ions. There is a method for rapid synthesis of GSH-protected CuNCs by ultrasonic treatment. GSH was mixed with Cu(II) ions in an aqueous solution, and the pH was adjusted to 6.0 using NaOH, followed by ultrasonic treatment for 15 minutes. The red-emitting fluorescent CuNCs were obtained after purification. Structural and optical analysis showed that the high density enhanced the co-affinity Cu(I)···Cu(I) interaction between and within NCs, and inhibited the intramolecular vibration and rotation of the ligand. The self-assembly strategy also allows the regularity of CuNCs in the component to be adjusted, resulting in polymorphic CuNCs components with various emission colors.[37]
Properties
Magnetic properties
Most atoms in a nanocluster are surface atoms. Thus, it is expected that the magnetic moment of an atom in a cluster will be larger than that of one in a bulk material. Lower coordination, lower dimensionality, and increasing interatomic distance in metal clusters contribute to enhancement of the magnetic moment in nanoclusters. Metal nanoclusters also show change in magnetic properties. For example, vanadium and rhodium are paramagnetic in bulk but become ferromagnetic in nanoclusters. Also, manganese is antiferromagnetic in bulk but ferromagnetic in nanoclusters. A small nanocluster is a nanomagnet, which can be made nonmagnetic simply by changing its structure. So they can form the basis of a nanomagnetic switch.[8][13]
Reactivity properties
Large surface-to-volume ratios and low coordination of surface atoms are primary reasons for the unique reactivity of nanoclusters. Thus, nanoclusters are widely used as catalysts.[16] The binding of ligands, their activation and reactions can be studied size-selectively by infrared spectroscopy.[38] Gold nanoclusters are an excellent example of a catalyst. While bulk gold is chemically inert, it becomes highly reactive when scaled down to nanometer scale. One of the properties that govern cluster reactivity is electron affinity. Chlorine has highest electron affinity of any material in the periodic table. Clusters can have high electron affinity and nanoclusters with high electron affinity are classified as super halogens. Super halogens are metal atoms at the core surrounded by halogen atoms.[8][13]
Optical properties
The optical properties of materials are determined by their electronic structure and band gap. The energy gap between the highest occupied molecular orbital and lowest unoccupied molecular orbital (HOMO/LUMO) varies with the size and composition of a nanocluster. Thus, the optical properties of nanoclusters change. Furthermore, the gaps can be modified by coating the nanoclusters with different ligands or surfactants. It is also possible to design nanoclusters with tailored band gaps and thus tailor optical properties by simply tuning the size and coating layer of the nanocluster.[3][6][8][13]
Applications
Nanoclusters potentially have many areas of application as they have unique optical, electrical, magnetic and reactivity properties. Nanoclusters are biocompatible, ultrasmall, and exhibit bright emission, hence promising candidates for fluorescence bio imaging or cellular labeling. Nanoclusters along with fluorophores are widely used for staining cells for study both in vitro and in vivo. Furthermore, nanoclusters can be used for sensing and detection applications.[39] They are able to detect copper and mercury ions in an aqueous solution based on fluorescence quenching. Also many small molecules, biological entities such as biomolecules, proteins, DNA, and RNA can be detected using nanoclusters. The unique reactivity properties and the ability to control the size and number of atoms in nanoclusters have proven to be a valuable method for increasing activity and tuning the selectivity in a catalytic process. Also since nanoparticles are magnetic materials and can be embedded in glass these nanoclusters can be used in optical data storage that can be used for many years without any loss of data.[3][6][7][8][9]
Major families of cluster compounds
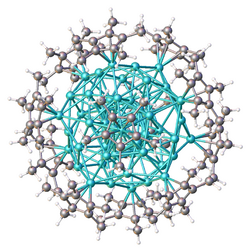
There is an infinite variety of compounds whose molecules are atom clusters or have such cluster at their core. Below are some classes that have received substantial attention from researchers.
Metallocarbohedrynes
Metallocarbohedrynes (or met-car for short) are a family of clusters with molecular formula M8C12, where M is a transition metal such as titanium, vanadium, zirconium, niobium, hafnium, molybdenum, chromium, or iron. They can be generated by vaporizing the desired metal with a laser, in an atmosphere containing the suitable hydrocarbon. They have been also detected, at a concentration of 1% or less, in the soot generated by an electric arc between two Ti-C electrodes. They feature metals atoms at the corners of a cube, but with the carbon atoms pushed inwards so as to be nearly coplanar with the faces of that cube.
Zintl clusters
Zintl compounds feature naked anionic clusters that are generated by reduction of heavy main group p elements, mostly metals or semimetals, with alkali metals, often as a solution in anhydrous liquid ammonia or ethylenediamine.[41] Examples of Zintl anions are [Bi3]3−, [Sn9]4−, [Pb9]4−, and [Sb7]3−.[42] Although these species are called "naked clusters", they are usually strongly associated with alkali metal cations. Some examples have been isolated using cryptate complexes of the alkali metal cation, e.g., [Pb10]2− anion, which features a capped square antiprismatic shape.[43] According to Wade's rules (2n+2) the number of cluster electrons is 22 and therefore a closo cluster. The compound is prepared from oxidation of K4Pb9 [44] by Au+ in PPh3AuCl (by reaction of tetrachloroauric acid and triphenylphosphine) in ethylene diamine with 2.2.2-crypt. This type of cluster was already known as is the endohedral [Ni@Pb10]2− (the cage contains one nickel atom). The icosahedral tin cluster [Sn12]2− or stannaspherene anion is another closed shell structure observed (but not isolated) with photoelectron spectroscopy.[45][46] With an internal diameter of 6.1 Ångstrom, it is of comparable size to fullerene and should be capable of containing small atoms in the same manner as endohedral fullerenes, and indeed exists a Sn12 cluster that contains an Ir atom: [Ir@Sn12]3−.[47]
See also
- Cluster
- Superatom
- Water molecules form clusters as well: see water clusters
- Metallaprism
- Paolo Chini
- Metal carbonyl cluster
Further reading (reviews)
- Schnöckel, Hansgeorg (2010). "Structures and Properties of Metalloid al and Ga Clusters Open Our Eyes to the Diversity and Complexity of Fundamental Chemical and Physical Processes during Formation and Dissolution of Metals". Chemical Reviews 110 (7): 4125–4163. doi:10.1021/cr900375g. PMID 20540559.
- Yano, Junko; Yachandra, Vittal (2014). "Mn4Ca Cluster in Photosynthesis: Where and How Water is Oxidized to Dioxygen". Chemical Reviews 114 (8): 4175–4205. doi:10.1021/cr4004874. PMID 24684576.
- Dermota, T. E.; Zhong, Q.; Castleman, A. W. (2004). "Ultrafast Dynamics in Cluster Systems". Chemical Reviews 104 (4): 1861–1886. doi:10.1021/cr020665e. PMID 15080714.
- Niedner-Schatteburg, Gereon; Bondybey, Vladimir E. (2000). "FT-ICR Studies of Solvation Effects in Ionic Water Cluster Reactions". Chemical Reviews 100 (11): 4059–4086. doi:10.1021/cr990065o. PMID 11749340.
- Gabriel, Jean-Christophe P.; Boubekeur, Kamal; Uriel, Santiago; Batail, Patrick (2001). "Chemistry of Hexanuclear Rhenium Chalcohalide Clusters". Chemical Reviews 101 (7): 2037–2066. doi:10.1021/cr980058k. PMID 11710240.
- Rohmer, Marie-Madeleine; Bénard, Marc; Poblet, Josep-M. (2000). "Structure, Reactivity, and Growth Pathways of Metallocarbohedrenes M8C12and Transition Metal/Carbon Clusters and Nanocrystals: A Challenge to Computational Chemistry". Chemical Reviews 100 (2): 495–542. doi:10.1021/cr9803885. PMID 11749244.
- Muetterties, E. L.; Rhodin, T. N.; Band, Elliot.; Brucker, C. F.; Pretzer, W. R. (1979). "Clusters and Surfaces". Chemical Reviews 79 (2): 91–137. doi:10.1021/cr60318a001.
- Chakraborty, Indranath; Pradeep, Thalappil (6 June 2017). "Atomically Precise Clusters of Noble Metals: Emerging Link between Atoms and Nanoparticles". Chemical Reviews 117 (12): 8208–8271. doi:10.1021/acs.chemrev.6b00769. PMID 28586213.
- Tanaka S. I; Miyazaki J; Tiwari D. K.; Jin T; Inouye Y. (2011). "Fluorescent Platinum Nanoclusters: Synthesis, Purification, Characterization, and Application to Bioimaging". Angewandte Chemie International Edition 50 (2): 431–435. doi:10.1002/anie.201004907. PMID 21154543.
- Karimi N; Kunwar P; Hassinen J; Ras R. H. A; Toivonen J (2016). "Micropatterning of silver nanoclusters embedded in polyvinyl alcohol films". Optics Letters 41 (15): 3627–3630. doi:10.1364/ol.41.003627. PMID 27472635. Bibcode: 2016OptL...41.3627K. https://aaltodoc.aalto.fi/handle/123456789/32811.
References
- ↑ Gary, Dylan C.; Flowers, Sarah E.; Kaminsky, Werner; Petrone, Alessio; Li, Xiaosong; Cossairt, Brandi M. (2016-02-10). "Single-Crystal and Electronic Structure of a 1.3 nm Indium Phosphide Nanocluster". Journal of the American Chemical Society 138 (5): 1510–1513. doi:10.1021/jacs.5b13214. ISSN 0002-7863. PMID 26784649. https://doi.org/10.1021/jacs.5b13214.
- ↑ 2.0 2.1 2.2 2.3 Kunwar, P; Hassinen, J; Bautista, G; Ras, R. H. A.; Toivonen, J (2016). "Sub-micron scale patterning of fluorescent silver nanoclusters using low-power laser". Scientific Reports 6: 23998. doi:10.1038/srep23998. PMID 27045598. Bibcode: 2016NatSR...623998K.
- ↑ 3.0 3.1 3.2 3.3 Kunwar, P; Hassinen, J; Bautista, G; Ras, R. H. A.; Toivonen, J (2014). "Direct Laser Writing of Photostable Fluorescent Silver Nanoclusters in Polymer Films". ACS Nano 8 (11): 11165–11171. doi:10.1021/nn5059503. PMID 25347726. https://aaltodoc.aalto.fi/handle/123456789/26539.
- ↑ Beecher, Alexander N.; Yang, Xiaohao; Palmer, Joshua H.; LaGrassa, Alexandra L.; Juhas, Pavol; Billinge, Simon J. L.; Owen, Jonathan S. (2014-07-30). "Atomic Structures and Gram Scale Synthesis of Three Tetrahedral Quantum Dots". Journal of the American Chemical Society 136 (30): 10645–10653. doi:10.1021/ja503590h. ISSN 0002-7863. PMID 25003618. https://doi.org/10.1021/ja503590h.
- ↑ Gary, Dylan C.; Terban, Maxwell W.; Billinge, Simon J. L.; Cossairt, Brandi M. (2015-02-24). "Two-Step Nucleation and Growth of InP Quantum Dots via Magic-Sized Cluster Intermediates". Chemistry of Materials 27 (4): 1432–1441. doi:10.1021/acs.chemmater.5b00286. ISSN 0897-4756. https://doi.org/10.1021/acs.chemmater.5b00286.
- ↑ 6.00 6.01 6.02 6.03 6.04 6.05 6.06 6.07 6.08 6.09 6.10 6.11 6.12 Dıez, I; Ras. R. H. (2011). "Fluorescent silver nanoclusters". Nanoscale 3 (5): 1963–70. doi:10.1039/c1nr00006c. PMID 21409225. Bibcode: 2011Nanos...3.1963D.
- ↑ 7.0 7.1 7.2 7.3 7.4 7.5 7.6 7.7 Zheng, J; Nicovich, P. R; Dickson, R. M. (2007). "Highly Fluorescent Noble Metal Quantum Dots". Annual Review of Physical Chemistry C 58: 409–431. doi:10.1146/annurev.physchem.58.032806.104546. PMID 17105412. Bibcode: 2007ARPC...58..409Z.
- ↑ 8.0 8.1 8.2 8.3 8.4 8.5 8.6 8.7 8.8 Wilcoxon, J. P; Abrams, B. L. (2006). "Synthesis, Structure and Properties of Metal Nanoclusters". Chemical Society Reviews 35 (11): 1162–1194. doi:10.1039/b517312b. PMID 17057844.
- ↑ 9.0 9.1 9.2 9.3 9.4 9.5 Shang, L; Dong, S; Nienhaus, G. U. (2011). "Ultra-Small Fluorescent Metal Nanoclusters: Synthesis and Biological Applications". Nano Today 6 (4): 401–418. doi:10.1016/j.nantod.2011.06.004.
- ↑ 10.0 10.1 10.2 Ashenfelter, B. A.; Desireddy, A; Yau, S. H; Goodson T; Bigioni, T. P (2015). "Fluorescence from Molecular Silver Nanoparticles". Journal of Physical Chemistry C 119 (35): 20728–20734. doi:10.1021/acs.jpcc.5b05735.
- ↑ Bhattarai, B; Zaker, Y; Atnagulov A; Yoon, B; Landman, U; Bigioni T. P. (2018). "Chemistry and Structure of Silver Molecular Nanoparticles". Accounts of Chemical Research 51 (12): 3104–3113. doi:10.1021/acs.accounts.8b00445. PMID 30462479.
- ↑ Bhattarai, B; Zaker, Y; Atnagulov A; Yoon, B; Landman, U; Bigioni T. P. (2018). "Chemistry and Structure of Silver Molecular Nanoparticles". Accounts of Chemical Research 51 (12): 3104–3113. doi:10.1021/acs.accounts.8b00445. PMID 30462479.
- ↑ 13.0 13.1 13.2 13.3 13.4 13.5 Jena, P; Castleman A. W. Jr. (2010). Nanoclusters. Elsevier. ISBN 9780444534408.
- ↑ 14.0 14.1 Chakraborty, I; Govindarajan, A; Erusappan, J; Ghosh, A; Pradeep, T; Yoon, B; Whetten, R. L.; Landman, U. (2012). "The Superstable 25 kDa Monolayer Protected Silver Nanoparticle: Measurements and Interpretation as an Icosahedral Ag152(SCH2CH2Ph)60 Cluster". Nano Letters 12 (11): 5861–5866. doi:10.1021/nl303220x. PMID 23094944. Bibcode: 2012NanoL..12.5861C.
- ↑ 15.0 15.1 15.2 Petty, J. T.; Story, S. P.; Hsiang, J. C.; Dickson, R. (2013). "DNA-Templated Molecular Silver Fluorophores". Journal of Physical Chemistry Letters 4 (7): 1148–1155. doi:10.1021/jz4000142. PMID 23745165.
- ↑ 16.0 16.1 16.2 Xu, H.; Suslick, K. S. (2010). "Sonochemical Synthesis of Highly Fluorescent Silver Nanoclusters". ACS Nano 4 (6): 3209–3214. doi:10.1021/nn100987k. PMID 20507161.
- ↑ 17.0 17.1 Gonzáles, B. S.; Blanco, M. C.; López-Quintela, A (2012). "Single step electro-chemical synthesis of hydrophilic/hydrophobic Ag5 and Ag6 blue luminescent clusters". Nanoscale 4 (24): 7632–7635. doi:10.1039/c2nr31994b. PMID 23064311. Bibcode: 2012Nanos...4.7632G.
- ↑ Conn, B. E.; Desireddy, A; Atnagulov, A; Wickramasinghe, S; Bhattarai, B; Yoon, B; Barnett, R. N.; Abdollahian, Y et al. (2015). "M4Ag44(p-MBA)30 Molecular Nanoparticles". Journal of Physical Chemistry C 119 (20): 11238–11249. doi:10.1021/jp512237b.
- ↑ Inorganic Chemistry Huheey, JE, 3rd ed. Harper and Row, New York
- ↑ Mingos, D. M. P.; Wales, D. J. (1990). Introduction to cluster chemistry. Englewood Cliffs, N.J: Prentice Hall. ISBN 0134743059. https://archive.org/details/introductiontocl0000ming.
- ↑ Lindsjö, Andreas Fischer, Martin; Kloo, Lars (2005-02-01). "Improvements of and Insights into the Isolation of Bismuth Polycations from Benzene Solution – Single-Crystal Structure Determinations of Bi8[GaCl4]2 and Bi5[GaCl4]3" (in en). European Journal of Inorganic Chemistry 2005 (4): 670–675. doi:10.1002/ejic.200400466. ISSN 1099-0682.
- ↑ Campbell, E. K.; Holz, M; Gerlich D; Maier, J. P. (2015). "Laboratory confirmation of C60+ as carrier of two diffuse interstellar bands". Nature 523 (7560): 322–325. doi:10.1038/nature14566. PMID 26178962. Bibcode: 2015Natur.523..322C.
- ↑ Rohlfing, Eric A; Cox, D. M; Kaldor, A (1984). "Production and characterization of supersonic carbon cluster beams". Journal of Chemical Physics 81 (7): 3322. doi:10.1063/1.447994. Bibcode: 1984JChPh..81.3322R.
- ↑ Kroto, H. W.; Heath, J. R.; O'Brien, S. C.; Curl, R. F.; Smalley, R. E. (1985). "C60: Buckminsterfullerene". Nature 318 (6042): 162–163. doi:10.1038/318162a0. Bibcode: 1985Natur.318..162K.
- ↑ Kubo, R (1962). "Electronic properties of metallic fine particles". Journal of the Physical Society of Japan 17 (6): 975. doi:10.1143/JPSJ.17.975. Bibcode: 1962JPSJ...17..975K.
- ↑ 26.0 26.1 Kumar, S (2013). Synthesis, Characterization and Application of Water- soluble Gold and Silver Nanoclusters (Ph. D. dissertation). Pittsburgh: Carnegie Mellon University.
- ↑ Schulz, Christopher; Daniels, Jörg; Bredow, Thomas; Beck, Johannes (2016). "The Electrochemical Synthesis of Polycationic Clusters". Angewandte Chemie International Edition 55 (3): 1173–1177. doi:10.1002/anie.201507644. PMID 26632775.
- ↑ Ott, Lisa Starkey; Finke, Richard G. (2007-05-01). "Transition-metal nanocluster stabilization for catalysis: A critical review of ranking methods and putative stabilizers" (in en). Coordination Chemistry Reviews 251 (9): 1075–1100. doi:10.1016/j.ccr.2006.08.016. ISSN 0010-8545. https://www.sciencedirect.com/science/article/pii/S0010854506002578.
- ↑ Brack, M (1993). "The physics of simple metal clusters: self-consistent jellium model and semiclassical approaches". Rev. Mod. Phys. 65 (3): 677. doi:10.1103/RevModPhys.65.677. Bibcode: 1993RvMP...65..677B. https://epub.uni-regensburg.de/11981/1/3.pdf.
- ↑ Hassinen, J. (2016). Noble Metal Nanoparticles and Clusters (Ph. D. dissertation). Espoo: Aalto University.
- ↑ Walter, M; Akola, J; Lopez-Aceved, O; Jadzinsky, P. D.; Calero, G; Ackerson, C. J.; Whetten, R. L.; Grönbeck, H. et al. (2008). "Unified View of Ligand-Protected Gold Clusters as Superatom Complexes". Proc. Natl. Acad. Sci. (U. S. A.) 105 (27): 9157–9162. doi:10.1073/pnas.0801001105. PMID 18599443. Bibcode: 2008PNAS..105.9157W.
- ↑ "Structure determination of isolated metal clusters via far-infrared spectroscopy". Phys. Rev. Lett. 93 (2): 023401. 2004. doi:10.1103/PhysRevLett.93.023401. PMID 15323913. Bibcode: 2004PhRvL..93b3401F. http://pubman.mpdl.mpg.de/pubman/item/escidoc:739325/component/escidoc:1477675/e023401.pdf.
- ↑ Heer, W. A (1993). "The physics of simple metal clusters: experimental aspects and simple models". Rev. Mod. Phys. 65 (3): 611. doi:10.1103/RevModPhys.65.611. Bibcode: 1993RvMP...65..611D.
- ↑ Kunwar, P; Turquet, L; Hassinen, J; Ras, R. H. A; Toivonen, J; Bautista, G (2016). "Holographic patterning of fluorescent microstructures comprising silver nanoclusters". Optical Materials Express 6 (3): 946–951. doi:10.1364/ome.6.000946. Bibcode: 2016OMExp...6..946K. https://aaltodoc.aalto.fi/handle/123456789/30968.
- ↑ Bellec, M; Royon, A; Bourhis, K; Choi, J; Bousquet, B; Treguer, M; Cardinal, T; Videau, J. J et al. (2010). "3D Patterning at the Nanoscale of Fluorescent Emitters in Glass". Journal of Physical Chemistry C 114 (37): 15584–15588. doi:10.1021/jp104049e.
- ↑ Cremer, G. D.; Sels, B. F; Hotta, J; Roeffaers, M. B. J.; Bartholomeeusen, E; Coutino-Gonzales, E; Valtchev, V; De Vos, D, E et al. (2010). "Optical Encoding of Silver Zeolite Microcarriers". Advanced Materials 22 (9): 957–960. doi:10.1002/adma.200902937. PMID 20217819. Bibcode: 2010AdM....22..957D.
- ↑ Qing, Taiping; Zhang, Kaiwu; Qing, Zhihe; Wang, Xuan; Long, Caicheng; Zhang, Peng; Hu, Haizhi; Feng, Bo (2019-09-05). "Recent progress in copper nanocluster-based fluorescent probing: a review" (in en). Microchimica Acta 186 (10): 670. doi:10.1007/s00604-019-3747-4. ISSN 1436-5073. PMID 31489488. https://doi.org/10.1007/s00604-019-3747-4.
- ↑ Fielicke, André (2023). "Probing the binding and activation of small molecules by gas-phase transition metal clusters via IR spectroscopy" (in en). Chemical Society Reviews 52 (11): 3778–3841. doi:10.1039/D2CS00104G. ISSN 0306-0012. http://xlink.rsc.org/?DOI=D2CS00104G.
- ↑ Zhao, Yu; Zhou, Huangmei; Zhang, Sanjun; Xu, Jianhua (2019-11-27). "The synthesis of metal nanoclusters and their applications in bio-sensing and imaging". Methods and Applications in Fluorescence 8 (1): 012001. doi:10.1088/2050-6120/ab57e7. ISSN 2050-6120. PMID 31726445. https://doi.org/10.1088/2050-6120/ab57e7.
- ↑ Vollet, Jean; Hartig, Jens R.; Schnöckel, Hansgeorg (2004). "Al50C120H180: A Pseudofullerene Shell of 60 Carbon Atoms and 60 Methyl Groups Protecting a Cluster Core of 50 Aluminum Atoms". Angewandte Chemie International Edition 43 (24): 3186–3189. doi:10.1002/anie.200453754. PMID 15199573.
- ↑ S. Scharfe; F. Kraus; S. Stegmaier; A. Schier; T. F. Fässler (2011). "Homoatomic Zintl Ions, Cage Compounds, and Intermetalloid Clusters of Group 14 and Group 15 Elements". Angewandte Chemie International Edition 50 (16): 3630–3670. doi:10.1002/anie.201001630. PMID 21455921.
- ↑ Zintl Ions: Principles and Recent Developments, Book Series: Structure and Bonding. T. F. Fässler (Ed.), Volume 140, Springer, Heidelberg, 2011 doi:10.1007/978-3-642-21181-2
- ↑ A. Spiekermann; S. D. Hoffmann; T. F. Fässler (2006). "The Zintl Ion [Pb10]2−: A Rare Example of a Homoatomic closo Cluster". Angewandte Chemie International Edition 45 (21): 3459–3462. doi:10.1002/anie.200503916. PMID 16622888.
- ↑ itself made by heating elemental potassium and lead at 350°C
- ↑ Tin particles are generated as K+[Sn12]2− by laser evaporation from solid tin containing 15% potassium and isolated by mass spectrometer before analysis
- ↑ Li-Feng Cui; Xin Huang; Lei-Ming Wang; Dmitry Yu. Zubarev; Alexander I. Boldyrev; Jun Li; Lai-Sheng Wang (2006). "Sn2−12: Stannaspherene". J. Am. Chem. Soc. 128 (26): 8390–8391. doi:10.1021/ja062052f. PMID 16802791.
- ↑ J.-Q. Wang; S. Stegmaier; B. Wahl; T. F. Fässler (2010). "Step by Step Synthesis of the Endohedral Stannaspherene [Ir@Sn12]3− via the Capped Cluster Anion [Sn9Ir(COD)]3−". Chem. Eur. J. 16 (6): 3532–3552. doi:10.1002/chem.200902815. PMID 20077544.
External links
- http://cluster-science.net - scientific community portal for clusters, fullerenes, nanotubes, nanostructures, and similar small systems
![]() | Original source: https://en.wikipedia.org/wiki/Nanocluster.
Read more |