Biology:Human brain
Human brain | |
---|---|
![]() The human brain, obtained after an autopsy | |
![]() Human brain and skull | |
Details | |
Precursor | Neural tube |
System | Central nervous system |
Artery | Internal carotid arteries, vertebral arteries |
Vein | Internal jugular vein, internal cerebral veins; external veins: (superior, middle, and inferior cerebral veins), basal vein, and cerebellar veins |
Identifiers | |
Latin | encephalon |
Greek | ἐγκέφαλος (enképhalos)[1] |
Anatomical terminology |
The brain is the central organ of the human nervous system, and with the spinal cord makes up the central nervous system. The brain consists of the cerebrum, the brainstem and the cerebellum. It controls most of the activities of the body, processing, integrating, and coordinating the information it receives from the sense organs, and making decisions as to the instructions sent to the rest of the body. The brain is contained in, and protected by, the skull bones of the head.
The cerebrum, the largest part of the human brain, consists of two cerebral hemispheres. Each hemisphere has an inner core composed of white matter, and an outer surface – the cerebral cortex – composed of grey matter. The cortex has an outer layer, the neocortex, and an inner allocortex. The neocortex is made up of six neuronal layers, while the allocortex has three or four. Each hemisphere is conventionally divided into four lobes – the frontal, temporal, parietal, and occipital lobes. The frontal lobe is associated with executive functions including self-control, planning, reasoning, and abstract thought, while the occipital lobe is dedicated to vision. Within each lobe, cortical areas are associated with specific functions, such as the sensory, motor and association regions. Although the left and right hemispheres are broadly similar in shape and function, some functions are associated with one side, such as language in the left and visual-spatial ability in the right. The hemispheres are connected by commissural nerve tracts, the largest being the corpus callosum.
The cerebrum is connected by the brainstem to the spinal cord. The brainstem consists of the midbrain, the pons, and the medulla oblongata. The cerebellum is connected to the brainstem by three pairs of nerve tracts called cerebellar peduncles. Within the cerebrum is the ventricular system, consisting of four interconnected ventricles in which cerebrospinal fluid is produced and circulated. Underneath the cerebral cortex are several important structures, including the thalamus, the epithalamus, the pineal gland, the hypothalamus, the pituitary gland, and the subthalamus; the limbic structures, including the amygdalae and the hippocampi, the claustrum, the various nuclei of the basal ganglia, the basal forebrain structures, and the three circumventricular organs. Brain structures that are not on the midplane exist in pairs, so there are for example two hippocampi and two amygdalae. The cells of the brain include neurons and supportive glial cells. There are more than 86 billion neurons in the brain, and a more or less equal number of other cells. Brain activity is made possible by the interconnections of neurons and their release of neurotransmitters in response to nerve impulses. Neurons connect to form neural pathways, neural circuits, and elaborate network systems. The whole circuitry is driven by the process of neurotransmission.
The brain is protected by the skull, suspended in cerebrospinal fluid, and isolated from the bloodstream by the blood–brain barrier. However, the brain is still susceptible to damage, disease, and infection. Damage can be caused by trauma, or a loss of blood supply known as a stroke. The brain is susceptible to degenerative disorders, such as Parkinson's disease, dementias including Alzheimer's disease, and multiple sclerosis. Psychiatric conditions, including schizophrenia and clinical depression, are thought to be associated with brain dysfunctions. The brain can also be the site of tumours, both benign and malignant; these mostly originate from other sites in the body.
The study of the anatomy of the brain is neuroanatomy, while the study of its function is neuroscience. Numerous techniques are used to study the brain. Specimens from other animals, which may be examined microscopically, have traditionally provided much information. Medical imaging technologies such as functional neuroimaging, and electroencephalography (EEG) recordings are important in studying the brain. The medical history of people with brain injury has provided insight into the function of each part of the brain. Neuroscience research has expanded considerably, and research is ongoing.
In culture, the philosophy of mind has for centuries attempted to address the question of the nature of consciousness and the mind–body problem. The pseudoscience of phrenology attempted to localise personality attributes to regions of the cortex in the 19th century. In science fiction, brain transplants are imagined in tales such as the 1942 Donovan's Brain.
Structure
Gross anatomy
The adult human brain weighs on average about 1.2–1.4 kg (2.6–3.1 lb) which is about 2% of the total body weight,[2][3] with a volume of around 1260 cm3 in men and 1130 cm3 in women.[4] There is substantial individual variation,[4] with the standard reference range for men being 1,180–1,620 g (2.60–3.57 lb)[5] and for women 1,030–1,400 g (2.27–3.09 lb).[6]
The cerebrum, consisting of the cerebral hemispheres, forms the largest part of the brain and overlies the other brain structures.[7] The outer region of the hemispheres, the cerebral cortex, is grey matter, consisting of cortical layers of neurons. Each hemisphere is divided into four main lobes – the frontal lobe, parietal lobe, temporal lobe, and occipital lobe.[8] Three other lobes are included by some sources which are a central lobe, a limbic lobe, and an insular lobe.[9] The central lobe comprises the precentral gyrus and the postcentral gyrus and is included since it forms a distinct functional role.[9][10]
The brainstem, resembling a stalk, attaches to and leaves the cerebrum at the start of the midbrain area. The brainstem includes the midbrain, the pons, and the medulla oblongata. Behind the brainstem is the cerebellum (Latin: little brain).[7]
The cerebrum, brainstem, cerebellum, and spinal cord are covered by four[11] membranes called meninges. The membranes are the tough dura mater; the middle arachnoid mater and the more delicate inner pia mater. Between the arachnoid mater and the pia mater is the subarachnoid space and subarachnoid cisterns, which contain the cerebrospinal fluid.[12] The outermost membrane of the cerebral cortex is the basement membrane of the pia mater called the glia limitans and is an important part of the blood–brain barrier.[13] The living brain is very soft, having a gel-like consistency similar to soft tofu.[14] The cortical layers of neurons constitute much of the cerebral grey matter, while the deeper subcortical regions of myelinated axons, make up the white matter.[7] The white matter of the brain makes up about half of the total brain volume.[15]
Cerebrum
The cerebrum is the largest part of the brain and is divided into nearly symmetrical left and right hemispheres by a deep groove, the longitudinal fissure.[16] Asymmetry between the lobes is noted as a petalia.[17] The hemispheres are connected by five commissures that span the longitudinal fissure, the largest of these is the corpus callosum.[7] Each hemisphere is conventionally divided into four main lobes; the frontal lobe, parietal lobe, temporal lobe, and occipital lobe, named according to the skull bones that overlie them.[8] Each lobe is associated with one or two specialised functions though there is some functional overlap between them.[18] The surface of the brain is folded into ridges (gyri) and grooves (sulci), many of which are named, usually according to their position, such as the frontal gyrus of the frontal lobe or the central sulcus separating the central regions of the hemispheres. There are many small variations in the secondary and tertiary folds.[19]
The outer part of the cerebrum is the cerebral cortex, made up of grey matter arranged in layers. It is 2 to 4 millimetres (0.079 to 0.157 in) thick, and deeply folded to give a convoluted appearance.[20] Beneath the cortex is the cerebral white matter. The largest part of the cerebral cortex is the neocortex, which has six neuronal layers. The rest of the cortex is of allocortex, which has three or four layers.[21]
The cortex is mapped by divisions into about fifty different functional areas known as Brodmann's areas. These areas are distinctly different when seen under a microscope.[22] The cortex is divided into two main functional areas – a motor cortex and a sensory cortex.[23] The primary motor cortex, which sends axons down to motor neurons in the brainstem and spinal cord, occupies the rear portion of the frontal lobe, directly in front of the somatosensory area. The primary sensory areas receive signals from the sensory nerves and tracts by way of relay nuclei in the thalamus. Primary sensory areas include the visual cortex of the occipital lobe, the auditory cortex in parts of the temporal lobe and insular cortex, and the somatosensory cortex in the parietal lobe. The remaining parts of the cortex are called the association areas. These areas receive input from the sensory areas and lower parts of the brain and are involved in the complex cognitive processes of perception, thought, and decision-making.[24] The main functions of the frontal lobe are to control attention, abstract thinking, behaviour, problem-solving tasks, and physical reactions and personality.[25][26] The occipital lobe is the smallest lobe; its main functions are visual reception, visual-spatial processing, movement, and colour recognition.[25][26] There is a smaller occipital lobule in the lobe known as the cuneus. The temporal lobe controls auditory and visual memories, language, and some hearing and speech.[25]
The cerebrum contains the ventricles where the cerebrospinal fluid is produced and circulated. Below the corpus callosum is the septum pellucidum, a membrane that separates the lateral ventricles. Beneath the lateral ventricles is the thalamus and to the front and below is the hypothalamus. The hypothalamus leads on to the pituitary gland. At the back of the thalamus is the brainstem.[27]
The basal ganglia, also called basal nuclei, are a set of structures deep within the hemispheres involved in behaviour and movement regulation.[28] The largest component is the striatum, others are the globus pallidus, the substantia nigra and the subthalamic nucleus.[28] The striatum is divided into a ventral striatum, and dorsal striatum, subdivisions that are based upon function and connections. The ventral striatum consists of the nucleus accumbens and the olfactory tubercle whereas the dorsal striatum consists of the caudate nucleus and the putamen. The putamen and the globus pallidus lie separated from the lateral ventricles and thalamus by the internal capsule, whereas the caudate nucleus stretches around and abuts the lateral ventricles on their outer sides.[29] At the deepest part of the lateral sulcus between the insular cortex and the striatum is a thin neuronal sheet called the claustrum.[30]
Below and in front of the striatum are a number of basal forebrain structures. These include the nucleus basalis, diagonal band of Broca, substantia innominata, and the medial septal nucleus. These structures are important in producing the neurotransmitter, acetylcholine, which is then distributed widely throughout the brain. The basal forebrain, in particular the nucleus basalis, is considered to be the major cholinergic output of the central nervous system to the striatum and neocortex.[31]
Cerebellum
The cerebellum is divided into an anterior lobe, a posterior lobe, and the flocculonodular lobe.[32] The anterior and posterior lobes are connected in the middle by the vermis.[33] Compared to the cerebral cortex, the cerebellum has a much thinner outer cortex that is narrowly furrowed into numerous curved transverse fissures.[33] Viewed from underneath between the two lobes is the third lobe the flocculonodular lobe.[34] The cerebellum rests at the back of the cranial cavity, lying beneath the occipital lobes, and is separated from these by the cerebellar tentorium, a sheet of fibre.[35]
It is connected to the brainstem by three pairs of nerve tracts called cerebellar peduncles. The superior pair connects to the midbrain; the middle pair connects to the medulla, and the inferior pair connects to the pons.[33] The cerebellum consists of an inner medulla of white matter and an outer cortex of richly folded grey matter.[35] The cerebellum's anterior and posterior lobes appear to play a role in the coordination and smoothing of complex motor movements, and the flocculonodular lobe in the maintenance of balance[36] although debate exists as to its cognitive, behavioural and motor functions.[37]
Brainstem
The brainstem lies beneath the cerebrum and consists of the midbrain, pons and medulla. It lies in the back part of the skull, resting on the part of the base known as the clivus, and ends at the foramen magnum, a large opening in the occipital bone. The brainstem continues below this as the spinal cord,[38] protected by the vertebral column.
Ten of the twelve pairs of cranial nerves[lower-alpha 1] emerge directly from the brainstem.[38] The brainstem also contains many cranial nerve nuclei and nuclei of peripheral nerves, as well as nuclei involved in the regulation of many essential processes including breathing, control of eye movements and balance.[39][38] The reticular formation, a network of nuclei of ill-defined formation, is present within and along the length of the brainstem.[38] Many nerve tracts, which transmit information to and from the cerebral cortex to the rest of the body, pass through the brainstem.[38]
Microanatomy
The human brain is primarily composed of neurons, glial cells, neural stem cells, and blood vessels. Types of neuron include interneurons, pyramidal cells including Betz cells, motor neurons (upper and lower motor neurons), and cerebellar Purkinje cells. Betz cells are the largest cells (by size of cell body) in the nervous system.[40] The adult human brain is estimated to contain 86±8 billion neurons, with a roughly equal number (85±10 billion) of non-neuronal cells.[41] Out of these neurons, 16 billion (19%) are located in the cerebral cortex, and 69 billion (80%) are in the cerebellum.[3][41]
Types of glial cell are astrocytes (including Bergmann glia), oligodendrocytes, ependymal cells (including tanycytes), radial glial cells, microglia, and a subtype of oligodendrocyte progenitor cells. Astrocytes are the largest of the glial cells. They are stellate cells with many processes radiating from their cell bodies. Some of these processes end as perivascular end-feet on capillary walls.[42] The glia limitans of the cortex is made up of astrocyte foot processes that serve in part to contain the cells of the brain.[13]
Mast cells are white blood cells that interact in the neuroimmune system in the brain.[43] Mast cells in the central nervous system are present in a number of structures including the meninges;[43] they mediate neuroimmune responses in inflammatory conditions and help to maintain the blood–brain barrier, particularly in brain regions where the barrier is absent.[43][44] Mast cells serve the same general functions in the body and central nervous system, such as effecting or regulating allergic responses, innate and adaptive immunity, autoimmunity, and inflammation.[43] Mast cells serve as the main effector cell through which pathogens can affect the biochemical signaling that takes place between the gastrointestinal tract and the central nervous system.[45][46]
Some 400 genes are shown to be brain-specific. In all neurons, ELAVL3 is expressed, and in pyramidal neurons, NRGN and REEP2 are also expressed. GAD1 – essential for the biosynthesis of the neurotransmitter GABA – is expressed in interneurons. Proteins expressed in glial cells include astrocyte markers GFAP and S100B whereas myelin basic protein and the transcription factor OLIG2 are expressed in oligodendrocytes.[47]
Cerebrospinal fluid

Cerebrospinal fluid is a clear, colourless transcellular fluid that circulates around the brain in the subarachnoid space, in the ventricular system, and in the central canal of the spinal cord. It also fills some gaps in the subarachnoid space, known as subarachnoid cisterns.[48] The four ventricles, two lateral, a third, and a fourth ventricle, all contain a choroid plexus that produces cerebrospinal fluid.[49] The third ventricle lies in the midline and is connected to the lateral ventricles.[48] A single duct, the cerebral aqueduct between the pons and the cerebellum, connects the third ventricle to the fourth ventricle.[50] Three separate openings, the middle and two lateral apertures, drain the cerebrospinal fluid from the fourth ventricle to the cisterna magna, one of the major cisterns. From here, cerebrospinal fluid circulates around the brain and spinal cord in the subarachnoid space, between the arachnoid mater and pia mater.[48] At any one time, there is about 150mL of cerebrospinal fluid – most within the subarachnoid space. It is constantly being regenerated and absorbed, and is replaced about once every 5–6 hours.[48]
A glymphatic system has been described[51][52][53] as the lymphatic drainage system of the brain. The brain-wide glymphatic pathway includes drainage routes from the cerebrospinal fluid, and from the meningeal lymphatic vessels that are associated with the dural sinuses, and run alongside the cerebral blood vessels.[54][55] The pathway drains interstitial fluid from the tissue of the brain.[55]
Blood supply
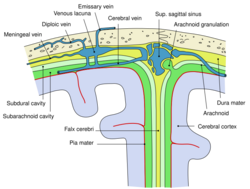
The internal carotid arteries supply oxygenated blood to the front of the brain and the vertebral arteries supply blood to the back of the brain.[56] These two circulations join in the circle of Willis, a ring of connected arteries that lies in the interpeduncular cistern between the midbrain and pons.[57]
The internal carotid arteries are branches of the common carotid arteries. They enter the cranium through the carotid canal, travel through the cavernous sinus and enter the subarachnoid space.[58] They then enter the circle of Willis, with two branches, the anterior cerebral arteries emerging. These branches travel forward and then upward along the longitudinal fissure, and supply the front and midline parts of the brain.[59] One or more small anterior communicating arteries join the two anterior cerebral arteries shortly after they emerge as branches.[59] The internal carotid arteries continue forward as the middle cerebral arteries. They travel sideways along the sphenoid bone of the eye socket, then upwards through the insula cortex, where final branches arise. The middle cerebral arteries send branches along their length.[58]
The vertebral arteries emerge as branches of the left and right subclavian arteries. They travel upward through transverse foramina which are spaces in the cervical vertebrae. Each side enters the cranial cavity through the foramen magnum along the corresponding side of the medulla.[58] They give off one of the three cerebellar branches. The vertebral arteries join in front of the middle part of the medulla to form the larger basilar artery, which sends multiple branches to supply the medulla and pons, and the two other anterior and superior cerebellar branches.[60] Finally, the basilar artery divides into two posterior cerebral arteries. These travel outwards, around the superior cerebellar peduncles, and along the top of the cerebellar tentorium, where it sends branches to supply the temporal and occipital lobes.[60] Each posterior cerebral artery sends a small posterior communicating artery to join with the internal carotid arteries.
Blood drainage
Cerebral veins drain deoxygenated blood from the brain. The brain has two main networks of veins: an exterior or superficial network, on the surface of the cerebrum that has three branches, and an interior network. These two networks communicate via anastomosing (joining) veins.[61] The veins of the brain drain into larger cavities of the dural venous sinuses usually situated between the dura mater and the covering of the skull.[62] Blood from the cerebellum and midbrain drains into the great cerebral vein. Blood from the medulla and pons of the brainstem have a variable pattern of drainage, either into the spinal veins or into adjacent cerebral veins.[61]
The blood in the deep part of the brain drains, through a venous plexus into the cavernous sinus at the front, and the superior and inferior petrosal sinuses at the sides, and the inferior sagittal sinus at the back.[62] Blood drains from the outer brain into the large superior sagittal sinus, which rests in the midline on top of the brain. Blood from here joins with blood from the straight sinus at the confluence of sinuses.[62]
Blood from here drains into the left and right transverse sinuses.[62] These then drain into the sigmoid sinuses, which receive blood from the cavernous sinus and superior and inferior petrosal sinuses. The sigmoid drains into the large internal jugular veins.[62][61]
The blood–brain barrier
The larger arteries throughout the brain supply blood to smaller capillaries. These smallest of blood vessels in the brain, are lined with cells joined by tight junctions and so fluids do not seep in or leak out to the same degree as they do in other capillaries; this creates the blood–brain barrier.[44] Pericytes play a major role in the formation of the tight junctions.[63] The barrier is less permeable to larger molecules, but is still permeable to water, carbon dioxide, oxygen, and most fat-soluble substances (including anaesthetics and alcohol).[44] The blood-brain barrier is not present in the circumventricular organs—which are structures in the brain that may need to respond to changes in body fluids—such as the pineal gland, area postrema, and some areas of the hypothalamus.[44] There is a similar blood–cerebrospinal fluid barrier, which serves the same purpose as the blood–brain barrier, but facilitates the transport of different substances into the brain due to the distinct structural characteristics between the two barrier systems.[44][64]
Development
At the beginning of the third week of development, the embryonic ectoderm forms a thickened strip called the neural plate.[65] By the fourth week of development the neural plate has widened to give a broad cephalic end, a less broad middle part and a narrow caudal end. These swellings are known as the primary brain vesicles and represent the beginnings of the forebrain (prosencephalon), midbrain (mesencephalon), and hindbrain (rhombencephalon).[66][67]
Neural crest cells (derived from the ectoderm) populate the lateral edges of the plate at the neural folds. In the fourth week—during the neurulation stage—the neural folds close to form the neural tube, bringing together the neural crest cells at the neural crest.[68] The neural crest runs the length of the tube with cranial neural crest cells at the cephalic end and caudal neural crest cells at the tail. Cells detach from the crest and migrate in a craniocaudal (head to tail) wave inside the tube.[68] Cells at the cephalic end give rise to the brain, and cells at the caudal end give rise to the spinal cord.[69]
The tube flexes as it grows, forming the crescent-shaped cerebral hemispheres at the head. The cerebral hemispheres first appear on day 32.[70] Early in the fourth week, the cephalic part bends sharply forward in a cephalic flexure.[68] This flexed part becomes the forebrain (prosencephalon); the adjoining curving part becomes the midbrain (mesencephalon) and the part caudal to the flexure becomes the hindbrain (rhombencephalon). These areas are formed as swellings known as the three primary brain vesicles. In the fifth week of development five secondary brain vesicles have formed.[71] The forebrain separates into two vesicles – an anterior telencephalon and a posterior diencephalon. The telencephalon gives rise to the cerebral cortex, basal ganglia, and related structures. The diencephalon gives rise to the thalamus and hypothalamus. The hindbrain also splits into two areas – the metencephalon and the myelencephalon. The metencephalon gives rise to the cerebellum and pons. The myelencephalon gives rise to the medulla oblongata.[72] Also during the fifth week, the brain divides into repeating segments called neuromeres.[66][73] In the hindbrain these are known as rhombomeres.[74]
A characteristic of the brain is the cortical folding known as gyrification. For just over five months of prenatal development the cortex is smooth. By the gestational age of 24 weeks, the wrinkled morphology showing the fissures that begin to mark out the lobes of the brain is evident.[75] Why the cortex wrinkles and folds is not well-understood, but gyrification has been linked to intelligence and neurological disorders, and a number of gyrification theories have been proposed.[75] These theories include those based on mechanical buckling,[76][18] axonal tension,[77] and differential tangential expansion.[76] What is clear is that gyrification is not a random process, but rather a complex developmentally predetermined process which generates patterns of folds that are consistent between individuals and most species.[76][78]
The first groove to appear in the fourth month is the lateral cerebral fossa.[70] The expanding caudal end of the hemisphere has to curve over in a forward direction to fit into the restricted space. This covers the fossa and turns it into a much deeper ridge known as the lateral sulcus and this marks out the temporal lobe.[70] By the sixth month other sulci have formed that demarcate the frontal, parietal, and occipital lobes.[70] A gene present in the human genome (ARHGAP11B) may play a major role in gyrification and encephalisation.[79]
Function
Motor control
The frontal lobe is involved in reasoning, motor control, emotion, and language. It contains the motor cortex, which is involved in planning and coordinating movement; the prefrontal cortex, which is responsible for higher-level cognitive functioning; and Broca’s area, which is essential for language production.[80] The motor system of the brain is responsible for the generation and control of movement.[81] Generated movements pass from the brain through nerves to motor neurons in the body, which control the action of muscles. The corticospinal tract carries movements from the brain, through the spinal cord, to the torso and limbs.[82] The cranial nerves carry movements related to the eyes, mouth and face.
Gross movement – such as locomotion and the movement of arms and legs – is generated in the motor cortex, divided into three parts: the primary motor cortex, found in the precentral gyrus and has sections dedicated to the movement of different body parts. These movements are supported and regulated by two other areas, lying anterior to the primary motor cortex: the premotor area and the supplementary motor area.[83] The hands and mouth have a much larger area dedicated to them than other body parts, allowing finer movement; this has been visualised in a motor homunculus.[83] Impulses generated from the motor cortex travel along the corticospinal tract along the front of the medulla and cross over (decussate) at the medullary pyramids. These then travel down the spinal cord, with most connecting to interneurons, in turn connecting to lower motor neurons within the grey matter that then transmit the impulse to move to muscles themselves.[82] The cerebellum and basal ganglia, play a role in fine, complex and coordinated muscle movements.[84] Connections between the cortex and the basal ganglia control muscle tone, posture and movement initiation, and are referred to as the extrapyramidal system.[85]
Sensory
The sensory nervous system is involved with the reception and processing of sensory information. This information is received through the cranial nerves, through tracts in the spinal cord, and directly at centres of the brain exposed to the blood.[86] The brain also receives and interprets information from the special senses of vision, smell, hearing, and taste. Mixed motor and sensory signals are also integrated.[86]
From the skin, the brain receives information about fine touch, pressure, pain, vibration and temperature. From the joints, the brain receives information about joint position.[87] The sensory cortex is found just near the motor cortex, and, like the motor cortex, has areas related to sensation from different body parts. Sensation collected by a sensory receptor on the skin is changed to a nerve signal, that is passed up a series of neurons through tracts in the spinal cord. The dorsal column–medial lemniscus pathway contains information about fine touch, vibration and position of joints. The pathway fibres travel up the back part of the spinal cord to the back part of the medulla, where they connect with second-order neurons that immediately send fibres across the midline. These fibres then travel upwards into the ventrobasal complex in the thalamus where they connect with third-order neurons which send fibres up to the sensory cortex.[87] The spinothalamic tract carries information about pain, temperature, and gross touch. The pathway fibres travel up the spinal cord and connect with second-order neurons in the reticular formation of the brainstem for pain and temperature, and also terminate at the ventrobasal complex of the thalamus for gross touch.[88]
Vision is generated by light that hits the retina of the eye. Photoreceptors in the retina transduce the sensory stimulus of light into an electrical nerve signal that is sent to the visual cortex in the occipital lobe. Visual signals leave the retinas through the optic nerves. Optic nerve fibres from the retinas' nasal halves cross to the opposite sides joining the fibres from the temporal halves of the opposite retinas to form the optic tracts. The arrangements of the eyes' optics and the visual pathways mean vision from the left visual field is received by the right half of each retina, is processed by the right visual cortex, and vice versa. The optic tract fibres reach the brain at the lateral geniculate nucleus, and travel through the optic radiation to reach the visual cortex.[89]
Hearing and balance are both generated in the inner ear. Sound results in vibrations of the ossicles which continue finally to the hearing organ, and change in balance results in movement of liquids within the inner ear. This creates a nerve signal that passes through the vestibulocochlear nerve. From here, it passes through to the cochlear nuclei, the superior olivary nucleus, the medial geniculate nucleus, and finally the auditory radiation to the auditory cortex.[90]
The sense of smell is generated by receptor cells in the epithelium of the olfactory mucosa in the nasal cavity. This information passes via the olfactory nerve which goes into the skull through a relatively permeable part. This nerve transmits to the neural circuitry of the olfactory bulb from where information is passed to the olfactory cortex.[91][92] Taste is generated from receptors on the tongue and passed along the facial and glossopharyngeal nerves into the solitary nucleus in the brainstem. Some taste information is also passed from the pharynx into this area via the vagus nerve. Information is then passed from here through the thalamus into the gustatory cortex.[93]
Regulation
Autonomic functions of the brain include the regulation, or rhythmic control of the heart rate and rate of breathing, and maintaining homeostasis.
Blood pressure and heart rate are influenced by the vasomotor centre of the medulla, which causes arteries and veins to be somewhat constricted at rest. It does this by influencing the sympathetic and parasympathetic nervous systems via the vagus nerve.[94] Information about blood pressure is generated by baroreceptors in aortic bodies in the aortic arch, and passed to the brain along the afferent fibres of the vagus nerve. Information about the pressure changes in the carotid sinus comes from carotid bodies located near the carotid artery and this is passed via a nerve joining with the glossopharyngeal nerve. This information travels up to the solitary nucleus in the medulla. Signals from here influence the vasomotor centre to adjust vein and artery constriction accordingly.[95]
The brain controls the rate of breathing, mainly by respiratory centres in the medulla and pons.[96] The respiratory centres control respiration, by generating motor signals that are passed down the spinal cord, along the phrenic nerve to the diaphragm and other muscles of respiration. This is a mixed nerve that carries sensory information back to the centres. There are four respiratory centres, three with a more clearly defined function, and an apneustic centre with a less clear function. In the medulla a dorsal respiratory group causes the desire to breathe in and receives sensory information directly from the body. Also in the medulla, the ventral respiratory group influences breathing out during exertion. In the pons the pneumotaxic centre influences the duration of each breath,[96] and the apneustic centre seems to have an influence on inhalation. The respiratory centres directly senses blood carbon dioxide and pH. Information about blood oxygen, carbon dioxide and pH levels are also sensed on the walls of arteries in the peripheral chemoreceptors of the aortic and carotid bodies. This information is passed via the vagus and glossopharyngeal nerves to the respiratory centres. High carbon dioxide, an acidic pH, or low oxygen stimulate the respiratory centres.[96] The desire to breathe in is also affected by pulmonary stretch receptors in the lungs which, when activated, prevent the lungs from overinflating by transmitting information to the respiratory centres via the vagus nerve.[96]
The hypothalamus in the diencephalon, is involved in regulating many functions of the body. Functions include neuroendocrine regulation, regulation of the circadian rhythm, control of the autonomic nervous system, and the regulation of fluid, and food intake. The circadian rhythm is controlled by two main cell groups in the hypothalamus. The anterior hypothalamus includes the suprachiasmatic nucleus and the ventrolateral preoptic nucleus which through gene expression cycles, generates a roughly 24 hour circadian clock. In the circadian day an ultradian rhythm takes control of the sleeping pattern. Sleep is an essential requirement for the body and brain and allows the closing down and resting of the body's systems. There are also findings that suggest that the daily build-up of toxins in the brain are removed during sleep.[97] Whilst awake the brain consumes a fifth of the body's total energy needs. Sleep necessarily reduces this use and gives time for the restoration of energy-giving ATP. The effects of sleep deprivation show the absolute need for sleep.[98]
The lateral hypothalamus contains orexinergic neurons that control appetite and arousal through their projections to the ascending reticular activating system.[99][100] The hypothalamus controls the pituitary gland through the release of peptides such as oxytocin, and vasopressin, as well as dopamine into the median eminence. Through the autonomic projections, the hypothalamus is involved in regulating functions such as blood pressure, heart rate, breathing, sweating, and other homeostatic mechanisms.[101] The hypothalamus also plays a role in thermal regulation, and when stimulated by the immune system, is capable of generating a fever. The hypothalamus is influenced by the kidneys: when blood pressure falls, the renin released by the kidneys stimulates a need to drink. The hypothalamus also regulates food intake through autonomic signals, and hormone release by the digestive system.[102]
Language
While language functions were traditionally thought to be localised to Wernicke's area and Broca's area,[103] it is now mostly accepted that a wider network of cortical regions contributes to language functions.[104][105][106]
The study on how language is represented, processed, and acquired by the brain is called neurolinguistics, which is a large multidisciplinary field drawing from cognitive neuroscience, cognitive linguistics, and psycholinguistics.[107]
Lateralisation
The cerebrum has a contralateral organisation with each hemisphere of the brain interacting primarily with one half of the body: the left side of the brain interacts with the right side of the body, and vice versa. This is theorized to be caused by a developmental axial twist.[108] Motor connections from the brain to the spinal cord, and sensory connections from the spinal cord to the brain, both cross sides in the brainstem. Visual input follows a more complex rule: the optic nerves from the two eyes come together at a point called the optic chiasm, and half of the fibres from each nerve split off to join the other.[109] The result is that connections from the left half of the retina, in both eyes, go to the left side of the brain, whereas connections from the right half of the retina go to the right side of the brain.[110] Because each half of the retina receives light coming from the opposite half of the visual field, the functional consequence is that visual input from the left side of the world goes to the right side of the brain, and vice versa.[111] Thus, the right side of the brain receives somatosensory input from the left side of the body, and visual input from the left side of the visual field.[112][113]
The left and right sides of the brain appear symmetrical, but they function asymmetrically.[114] For example, the counterpart of the left-hemisphere motor area controlling the right hand is the right-hemisphere area controlling the left hand. There are, however, several important exceptions, involving language and spatial cognition. The left frontal lobe is dominant for language. If a key language area in the left hemisphere is damaged, it can leave the victim unable to speak or understand,[114] whereas equivalent damage to the right hemisphere would cause only minor impairment to language skills.
A substantial part of current understanding of the interactions between the two hemispheres has come from the study of "split-brain patients"—people who underwent surgical transection of the corpus callosum in an attempt to reduce the severity of epileptic seizures.[115] These patients do not show unusual behaviour that is immediately obvious, but in some cases can behave almost like two different people in the same body, with the right hand taking an action and then the left hand undoing it.[115][116] These patients, when briefly shown a picture on the right side of the point of visual fixation, are able to describe it verbally, but when the picture is shown on the left, are unable to describe it, but may be able to give an indication with the left hand of the nature of the object shown.[116][117]
Emotion
Emotions are generally defined as two-step multicomponent processes involving elicitation, followed by psychological feelings, appraisal, expression, autonomic responses, and action tendencies.[118] Attempts to localise basic emotions to certain brain regions have been controversial; some research found no evidence for specific locations corresponding to emotions, but instead found circuitry involved in general emotional processes. The amygdala, orbitofrontal cortex, mid and anterior insula cortex and lateral prefrontal cortex, appeared to be involved in generating the emotions, while weaker evidence was found for the ventral tegmental area, ventral pallidum and nucleus accumbens in incentive salience.[119] Others, however, have found evidence of activation of specific regions, such as the basal ganglia in happiness, the subcallosal cingulate cortex in sadness, and amygdala in fear.[120]
Cognition
The brain is responsible for cognition,[121][122] which functions through numerous processes and executive functions.[122][123][124] Executive functions include the ability to filter information and tune out irrelevant stimuli with attentional control and cognitive inhibition, the ability to process and manipulate information held in working memory, the ability to think about multiple concepts simultaneously and switch tasks with cognitive flexibility, the ability to inhibit impulses and prepotent responses with inhibitory control, and the ability to determine the relevance of information or appropriateness of an action.[123][124] Higher order executive functions require the simultaneous use of multiple basic executive functions, and include planning, prospection and fluid intelligence (i.e., reasoning and problem solving).[124]
The prefrontal cortex plays a significant role in mediating executive functions.[122][124][125] Planning involves activation of the dorsolateral prefrontal cortex (DLPFC), anterior cingulate cortex, angular prefrontal cortex, right prefrontal cortex, and supramarginal gyrus.[125] Working memory manipulation involves the DLPFC, inferior frontal gyrus, and areas of the parietal cortex.[122][125] Inhibitory control involves multiple areas of the prefrontal cortex, as well as the caudate nucleus and subthalamic nucleus.[124][125][126]
Physiology
Neurotransmission
Brain activity is made possible by the interconnections of neurons that are linked together to reach their targets.[127] A neuron consists of a cell body, axon, and dendrites. Dendrites are often extensive branches that receive information in the form of signals from the axon terminals of other neurons. The signals received may cause the neuron to initiate an action potential (an electrochemical signal or nerve impulse) which is sent along its axon to the axon terminal, to connect with the dendrites or with the cell body of another neuron. An action potential is initiated at the initial segment of an axon, which contains a specialised complex of proteins.[128] When an action potential reaches the axon terminal it triggers the release of a neurotransmitter at a synapse that propagates a signal that acts on the target cell.[129] These chemical neurotransmitters include dopamine, serotonin, GABA, glutamate, and acetylcholine.[130] GABA is the major inhibitory neurotransmitter in the brain, and glutamate is the major excitatory neurotransmitter.[131] Neurons link at synapses to form neural pathways, neural circuits, and large elaborate network systems such as the salience network and the default mode network, and the activity between them is driven by the process of neurotransmission.
Metabolism
The brain consumes up to 20% of the energy used by the human body, more than any other organ.[132] In humans, blood glucose is the primary source of energy for most cells and is critical for normal function in a number of tissues, including the brain.[133] The human brain consumes approximately 60% of blood glucose in fasted, sedentary individuals.[133] Brain metabolism normally relies upon blood glucose as an energy source, but during times of low glucose (such as fasting, endurance exercise, or limited carbohydrate intake), the brain uses ketone bodies for fuel with a smaller need for glucose. The brain can also utilize lactate during exercise.[134] The brain stores glucose in the form of glycogen, albeit in significantly smaller amounts than that found in the liver or skeletal muscle.[135] Long-chain fatty acids cannot cross the blood–brain barrier, but the liver can break these down to produce ketone bodies. However, short-chain fatty acids (e.g., butyric acid, propionic acid, and acetic acid) and the medium-chain fatty acids, octanoic acid and heptanoic acid, can cross the blood–brain barrier and be metabolised by brain cells.[136][137][138]
Although the human brain represents only 2% of the body weight, it receives 15% of the cardiac output, 20% of total body oxygen consumption, and 25% of total body glucose utilization.[139] The brain mostly uses glucose for energy, and deprivation of glucose, as can happen in hypoglycemia, can result in loss of consciousness.[140] The energy consumption of the brain does not vary greatly over time, but active regions of the cortex consume somewhat more energy than inactive regions, which forms the basis for the functional neuroimaging methods of PET and fMRI.[141] These techniques provide a three-dimensional image of metabolic activity.[142] A preliminary study showed that brain metabolic requirements in humans peak at about five years old.[143]
The function of sleep is not fully understood; however, there is evidence that sleep enhances the clearance of metabolic waste products, some of which are potentially neurotoxic, from the brain and may also permit repair.[53][144][145] Evidence suggests that the increased clearance of metabolic waste during sleep occurs via increased functioning of the glymphatic system.[53] Sleep may also have an effect on cognitive function by weakening unnecessary connections.[146]
Research
The brain is not fully understood, and research is ongoing.[147] Neuroscientists, along with researchers from allied disciplines, study how the human brain works. The boundaries between the specialties of neuroscience, neurology and other disciplines such as psychiatry have faded as they are all influenced by basic research in neuroscience.
Neuroscience research has expanded considerably. The "Decade of the Brain", an initiative of the United States Government in the 1990s, is considered to have marked much of this increase in research,[148] and was followed in 2013 by the BRAIN Initiative.[149] The Human Connectome Project was a five-year study launched in 2009 to analyse the anatomical and functional connections of parts of the brain, and has provided much data.[147]
An emerging phase in research may be that of simulating brain activity.[150]
The chemistry of memory storage, logical thought processes, and conciousness are other areas of research. A hypothesis is that the brain stores memories through a construct of intermolecular and intramolecular network patterns of folded glycoproteins, and that memory may be encoded in hydrogen-bonding patterns.[151][152]
Methods
Information about the structure and function of the human brain comes from a variety of experimental methods, including animals and humans. Information about brain trauma and stroke has provided information about the function of parts of the brain and the effects of brain damage. Neuroimaging is used to visualise the brain and record brain activity. Electrophysiology is used to measure, record and monitor the electrical activity of the cortex. Measurements may be of local field potentials of cortical areas, or of the activity of a single neuron. An electroencephalogram can record the electrical activity of the cortex using electrodes placed non-invasively on the scalp.[153][154]
Invasive measures include electrocorticography, which uses electrodes placed directly on the exposed surface of the brain. This method is used in cortical stimulation mapping, used in the study of the relationship between cortical areas and their systemic function.[155] By using much smaller microelectrodes, single-unit recordings can be made from a single neuron that give a high spatial resolution and high temporal resolution. This has enabled the linking of brain activity to behaviour, and the creation of neuronal maps.[156]
The development of cerebral organoids has opened ways for studying the growth of the brain, and of the cortex, and for understanding disease development, offering further implications for therapeutic applications.[157][158]
Imaging
Functional neuroimaging techniques show changes in brain activity that relate to the function of specific brain areas. One technique is functional magnetic resonance imaging (fMRI) which has the advantages over earlier methods of SPECT and PET of not needing the use of radioactive materials and of offering a higher resolution.[159] Another technique is functional near-infrared spectroscopy. These methods rely on the haemodynamic response that shows changes in brain activity in relation to changes in blood flow, useful in mapping functions to brain areas.[160] Resting state fMRI looks at the interaction of brain regions whilst the brain is not performing a specific task.[161] This is also used to show the default mode network.
Any electrical current generates a magnetic field; neural oscillations induce weak magnetic fields, and in functional magnetoencephalography the current produced can show localised brain function in high resolution.[162] Tractography uses MRI and image analysis to create 3D images of the nerve tracts of the brain. Connectograms give a graphical representation of the neural connections of the brain.[163]
Differences in brain structure can be measured in some disorders, notably schizophrenia and dementia. Different biological approaches using imaging have given more insight for example into the disorders of depression and obsessive-compulsive disorder. A key source of information about the function of brain regions is the effects of damage to them.[164]
Advances in neuroimaging have enabled objective insights into mental disorders, leading to faster diagnosis, more accurate prognosis, and better monitoring.[165]
Gene and protein expression
Bioinformatics is a field of study that includes the creation and advancement of databases, and computational and statistical techniques, that can be used in studies of the human brain, particularly in the areas of gene and protein expression. Bioinformatics and studies in genomics, and functional genomics, generated the need for DNA annotation, a transcriptome technology, identifying genes, their locations and functions.[166][167][168] GeneCards is a major database.
(As of 2017), just under 20,000 protein-coding genes are seen to be expressed in the human,[166] and some 400 of these genes are brain-specific.[169][170] The data that has been provided on gene expression in the brain has fuelled further research into a number of disorders. The long term use of alcohol for example, has shown altered gene expression in the brain, and cell-type specific changes that may relate to alcohol use disorder.[171] These changes have been noted in the synaptic transcriptome in the prefrontal cortex, and are seen as a factor causing the drive to alcohol dependence, and also to other substance abuses.[172]
Other related studies have also shown evidence of synaptic alterations and their loss, in the ageing brain. Changes in gene expression alter the levels of proteins in various neural pathways and this has been shown to be evident in synaptic contact dysfunction or loss. This dysfunction has been seen to affect many structures of the brain and has a marked effect on inhibitory neurons resulting in a decreased level of neurotransmission, and subsequent cognitive decline and disease.[173][174]
Clinical significance
Injury
Injury to the brain can manifest in many ways. Traumatic brain injury, for example received in contact sport, after a fall, or a traffic or work accident, can be associated with both immediate and longer-term problems. Immediate problems may include bleeding within the brain, this may compress the brain tissue or damage its blood supply. Bruising to the brain may occur. Bruising may cause widespread damage to the nerve tracts that can lead to a condition of diffuse axonal injury.[175] A fractured skull, injury to a particular area, deafness, and concussion are also possible immediate developments. In addition to the site of injury, the opposite side of the brain may be affected, termed a contrecoup injury. Longer-term issues that may develop include posttraumatic stress disorder, and hydrocephalus. Chronic traumatic encephalopathy can develop following multiple head injuries.[176]
Disease
Neurodegenerative diseases result in progressive damage to different parts of the brain's function, and worsen with age. Common examples include dementia such as Alzheimer's disease, alcoholic dementia or vascular dementia; Parkinson's disease; and other rarer infectious, genetic, or metabolic causes such as Huntington's disease, motor neuron diseases, HIV dementia, syphilis-related dementia and Wilson's disease. Neurodegenerative diseases can affect different parts of the brain, and can affect movement, memory, and cognition.[177]
Cerebral atherosclerosis is atherosclerosis that affects the brain. It results from the build-up of plaques formed of cholesterol, in the large arteries of the brain, and can be mild to significant. When significant, arteries can become narrowed enough to reduce blood flow. It contributes to the development of dementia, and has protein similarities to those found in Alzheimer’s disease.[178]
The brain, although protected by the blood–brain barrier, can be affected by infections including viruses, bacteria and fungi. Infection may be of the meninges (meningitis), the brain matter (encephalitis), or within the brain matter (such as a cerebral abscess).[179] Rare prion diseases including Creutzfeldt–Jakob disease and its variant, and kuru may also affect the brain.[179]
Tumours
Brain tumours can be either benign or cancerous. Most malignant tumours arise from another part of the body, most commonly from the lung, breast and skin.[180] Cancers of brain tissue can also occur, and originate from any tissue in and around the brain. Meningioma, cancer of the meninges around the brain, is more common than cancers of brain tissue.[180] Cancers within the brain may cause symptoms related to their size or position, with symptoms including headache and nausea, or the gradual development of focal symptoms such as gradual difficulty seeing, swallowing, talking, or as a change of mood.[180] Cancers are in general investigated through the use of CT scans and MRI scans. A variety of other tests including blood tests and lumbar puncture may be used to investigate for the cause of the cancer and evaluate the type and stage of the cancer.[180] The corticosteroid dexamethasone is often given to decrease the swelling of brain tissue around a tumour. Surgery may be considered, however given the complex nature of many tumours or based on tumour stage or type, radiotherapy or chemotherapy may be considered more suitable.[180]
Mental disorders
Mental disorders, such as depression, schizophrenia, bipolar disorder, posttraumatic stress disorder, attention deficit hyperactivity disorder, obsessive-compulsive disorder, Tourette syndrome, and addiction, are known to relate to the functioning of the brain.[126][130][181] Treatment for mental disorders may include psychotherapy, psychiatry, social intervention and personal recovery work or cognitive behavioural therapy; the underlying issues and associated prognoses vary significantly between individuals.[182]
Epilepsy
Epileptic seizures are thought to relate to abnormal electrical activity.[183] Seizure activity can manifest as absence of consciousness, focal effects such as limb movement or impediments of speech, or be generalized in nature.[183] Status epilepticus refers to a seizure or series of seizures that have not terminated within 5 minutes.[184] Seizures have a large number of causes, however many seizures occur without a definitive cause being found. In a person with epilepsy, risk factors for further seizures may include sleeplessness, drug and alcohol intake, and stress. Seizures may be assessed using blood tests, EEG and various medical imaging techniques based on the medical history and medical examination findings.[183] In addition to treating an underlying cause and reducing exposure to risk factors, anticonvulsant medications can play a role in preventing further seizures.[183]
Congenital
Some brain disorders, such as Tay–Sachs disease,[185] are congenital and linked to genetic and chromosomal mutations.[186] A rare group of congenital cephalic disorders known as lissencephaly is characterised by the lack of, or inadequacy of, cortical folding.[187] Normal development of the brain can be affected during pregnancy by nutritional deficiencies,[188] teratogens,[189] infectious diseases,[190] and by the use of recreational drugs, including alcohol (which may result in fetal alcohol spectrum disorders).[188][191] Most cerebral arteriovenous malformations are congenital, these tangled networks of blood vessels may remain without symptoms but at their worst may rupture and cause intracranial hemorrhaging.[192]
Stroke

A stroke is a decrease in blood supply to an area of the brain causing cell death and brain injury. This can lead to a wide range of symptoms, including the "FAST" symptoms of facial droop, arm weakness, and speech difficulties (including with speaking and finding words or forming sentences).[193] Symptoms relate to the function of the affected area of the brain and can point to the likely site and cause of the stroke. Difficulties with movement, speech, or sight usually relate to the cerebrum, whereas imbalance, double vision, vertigo and symptoms affecting more than one side of the body usually relate to the brainstem or cerebellum.[194]
Most strokes result from loss of blood supply, typically because of an embolus, rupture of a fatty plaque causing thrombus, or narrowing of small arteries. Strokes can also result from bleeding within the brain.[195] Transient ischaemic attacks (TIAs) are strokes in which symptoms resolve within 24 hours.[195] Investigation into the stroke will involve a medical examination (including a neurological examination) and the taking of a medical history, focusing on the duration of the symptoms and risk factors (including high blood pressure, atrial fibrillation, and smoking).[196] Further investigation is needed in younger patients.[197] An ECG and biotelemetry may be conducted to identify atrial fibrillation; an ultrasound can investigate narrowing of the carotid arteries; an echocardiogram can be used to look for clots within the heart, diseases of the heart valves or the presence of a patent foramen ovale.[197] Blood tests are routinely done as part of the workup including diabetes tests and a lipid profile.[197]
Some treatments for stroke are time-critical. These include clot dissolution or surgical removal of a clot for ischaemic strokes, and decompression for haemorrhagic strokes.[198][199] As stroke is time critical,[200] hospitals and even pre-hospital care of stroke involves expedited investigations – usually a CT scan to investigate for a haemorrhagic stroke and a CT or MR angiogram to evaluate arteries that supply the brain.[197] MRI scans, not as widely available, may be able to demonstrate the affected area of the brain more accurately, particularly with ischaemic stroke.[197]
Having experienced a stroke, a person may be admitted to a stroke unit, and treatments may be directed as preventing future strokes, including ongoing anticoagulation (such as aspirin or clopidogrel), antihypertensives, and lipid-lowering drugs.[198] A multidisciplinary team including speech pathologists, physiotherapists, occupational therapists, and psychologists plays a large role in supporting a person affected by a stroke and their rehabilitation.[201][197] A history of stroke increases the risk of developing dementia by around 70%, and recent stroke increases the risk by around 120%.[202]
Brain death
Brain death refers to an irreversible total loss of brain function.[203][204] This is characterised by coma, loss of reflexes, and apnoea,[203] however, the declaration of brain death varies geographically and is not always accepted.[204] In some countries there is also a defined syndrome of brainstem death.[205] Declaration of brain death can have profound implications as the declaration, under the principle of medical futility, will be associated with the withdrawal of life support,[206] and as those with brain death often have organs suitable for organ donation.[204][207] The process is often made more difficult by poor communication with patients' families.[208]
When brain death is suspected, reversible differential diagnoses such as, electrolyte, neurological and drug-related cognitive suppression need to be excluded.[203][206] Testing for reflexes[lower-alpha 2] can be of help in the decision, as can the absence of response and breathing.[206] Clinical observations, including a total lack of responsiveness, a known diagnosis, and neural imaging evidence, may all play a role in the decision to pronounce brain death.[203]
Society and culture
Neuroanthropology is the study of the relationship between culture and the brain. It explores how the brain gives rise to culture, and how culture influences brain development.[209] Cultural differences and their relation to brain development and structure are researched in different fields.[210]
The mind
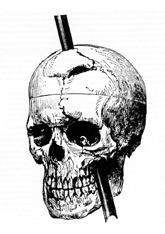
The philosophy of the mind studies such issues as the problem of understanding consciousness and the mind–body problem. The relationship between the brain and the mind is a significant challenge both philosophically and scientifically. This is because of the difficulty in explaining how mental activities, such as thoughts and emotions, can be implemented by physical structures such as neurons and synapses, or by any other type of physical mechanism. This difficulty was expressed by Gottfried Leibniz in the analogy known as Leibniz's Mill:
One is obliged to admit that perception and what depends upon it is inexplicable on mechanical principles, that is, by figures and motions. In imagining that there is a machine whose construction would enable it to think, to sense, and to have perception, one could conceive it enlarged while retaining the same proportions, so that one could enter into it, just like into a windmill. Supposing this, one should, when visiting within it, find only parts pushing one another, and never anything by which to explain a perception.
- — Leibniz, Monadology[212]
Doubt about the possibility of a mechanistic explanation of thought drove René Descartes, and most other philosophers along with him, to dualism: the belief that the mind is to some degree independent of the brain.[213] There has always, however, been a strong argument in the opposite direction. There is clear empirical evidence that physical manipulations of, or injuries to, the brain (for example by drugs or by lesions, respectively) can affect the mind in potent and intimate ways.[214][215] In the 19th century, the case of Phineas Gage, a railway worker who was injured by a stout iron rod passing through his brain, convinced both researchers and the public that cognitive functions were localised in the brain.[211] Following this line of thinking, a large body of empirical evidence for a close relationship between brain activity and mental activity has led most neuroscientists and contemporary philosophers to be materialists, believing that mental phenomena are ultimately the result of, or reducible to, physical phenomena.[216]
Brain size
The size of the brain and a person's intelligence are not strongly related.[217] Studies tend to indicate small to moderate correlations (averaging around 0.3 to 0.4) between brain volume and IQ.[218] The most consistent associations are observed within the frontal, temporal, and parietal lobes, the hippocampi, and the cerebellum, but these only account for a relatively small amount of variance in IQ, which itself has only a partial relationship to general intelligence and real-world performance.[219][220]
Other animals, including whales and elephants have larger brains than humans. However, when the brain-to-body mass ratio is taken into account, the human brain is almost twice as large as that of a bottlenose dolphin, and three times as large as that of a chimpanzee. However, a high ratio does not of itself demonstrate intelligence: very small animals have high ratios and the treeshrew has the largest quotient of any mammal.[221]
In popular culture
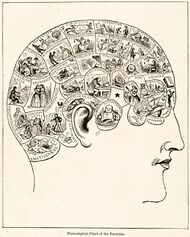
Earlier ideas about the relative importance of the different organs of the human body sometimes emphasised the heart.[222] Modern Western popular conceptions, in contrast, have placed increasing focus on the brain.[223]
Research has disproved some common misconceptions about the brain. These include both ancient and modern myths. It is not true (for example) that neurons are not replaced after the age of two; nor that normal humans use only ten per cent of the brain.[224] Popular culture has also oversimplified the lateralisation of the brain by suggesting that functions are completely specific to one side of the brain or the other. Akio Mori coined the term "game brain" for the unreliably supported theory that spending long periods playing video games harmed the brain's pre-frontal region, and impaired the expression of emotion and creativity.[225]
Historically, particularly in the early-19th century, the brain featured in popular culture through phrenology, a pseudoscience that assigned personality attributes to different regions of the cortex. The cortex remains important in popular culture as covered in books and satire.[226][227]
The human brain can feature in science fiction, with themes such as brain transplants and cyborgs (beings with features like partly artificial brains).[228] The 1942 science-fiction book (adapted three times for the cinema) Donovan's Brain tells the tale of an isolated brain kept alive in vitro, gradually taking over the personality of the book's protagonist.[229]
History
Early history
The Edwin Smith Papyrus, an ancient Egyptian medical treatise written in the 17th century BC, contains the earliest recorded reference to the brain. The hieroglyph for brain, occurring eight times in this papyrus, describes the symptoms, diagnosis, and prognosis of two traumatic injuries to the head. The papyrus mentions the external surface of the brain, the effects of injury (including seizures and aphasia), the meninges, and cerebrospinal fluid.[230][231]
In the fifth century BC, Alcmaeon of Croton in Magna Grecia, first considered the brain to be the seat of the mind.[231] Also in the fifth century BC in Athens, the unknown author of On the Sacred Disease, a medical treatise which is part of the Hippocratic Corpus and traditionally attributed to Hippocrates, believed the brain to be the seat of intelligence. Aristotle, in his biology initially believed the heart to be the seat of intelligence, and saw the brain as a cooling mechanism for the blood. He reasoned that humans are more rational than the beasts because, among other reasons, they have a larger brain to cool their hot-bloodedness.[232] Aristotle did describe the meninges and distinguished between the cerebrum and cerebellum.[233]
Herophilus of Chalcedon in the fourth and third centuries BC distinguished the cerebrum and the cerebellum, and provided the first clear description of the ventricles; and with Erasistratus of Ceos experimented on living brains. Their works are now mostly lost, and we know about their achievements due mostly to secondary sources. Some of their discoveries had to be re-discovered a millennium after their deaths.[231] Anatomist physician Galen in the second century AD, during the time of the Roman Empire, dissected the brains of sheep, monkeys, dogs, and pigs. He concluded that, as the cerebellum was denser than the brain, it must control the muscles, while as the cerebrum was soft, it must be where the senses were processed. Galen further theorised that the brain functioned by movement of animal spirits through the ventricles.[231][232]
Renaissance
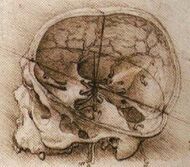
In 1316, Mondino de Luzzi's Anathomia began the modern study of brain anatomy.[234] Niccolò Massa discovered in 1536 that the ventricles were filled with fluid.[235] Archangelo Piccolomini of Rome was the first to distinguish between the cerebrum and cerebral cortex.[236] In 1543 Andreas Vesalius published his seven-volume De humani corporis fabrica.[236][237][238] The seventh book covered the brain and eye, with detailed images of the ventricles, cranial nerves, pituitary gland, meninges, structures of the eye, the vascular supply to the brain and spinal cord, and an image of the peripheral nerves.[239] Vesalius rejected the common belief that the ventricles were responsible for brain function, arguing that many animals have a similar ventricular system to humans, but no true intelligence.[236]
René Descartes proposed the theory of dualism to tackle the issue of the brain's relation to the mind. He suggested that the pineal gland was where the mind interacted with the body, serving as the seat of the soul and as the connection through which animal spirits passed from the blood into the brain.[235] This dualism likely provided impetus for later anatomists to further explore the relationship between the anatomical and functional aspects of brain anatomy.[240]
Thomas Willis is considered a second pioneer in the study of neurology and brain science. He wrote Cerebri Anatome (Latin: Anatomy of the brain)[lower-alpha 3] in 1664, followed by Cerebral Pathology in 1667. In these he described the structure of the cerebellum, the ventricles, the cerebral hemispheres, the brainstem, and the cranial nerves, studied its blood supply; and proposed functions associated with different areas of the brain.[236] The circle of Willis was named after his investigations into the blood supply of the brain, and he was the first to use the word "neurology."[241] Willis removed the brain from the body when examining it, and rejected the commonly held view that the cortex only consisted of blood vessels, and the view of the last two millennia that the cortex was only incidentally important.[236]
In the middle of 19th century Emil du Bois-Reymond and Hermann von Helmholtz were able to use a galvanometer to show that electrical impulses passed at measurable speeds along nerves, refuting the view of their teacher Johannes Peter Müller that the nerve impulse was a vital function that could not be measured.[242][243][244] Richard Caton in 1875 demonstrated electrical impulses in the cerebral hemispheres of rabbits and monkeys.[245] In the 1820s, Jean Pierre Flourens pioneered the experimental method of damaging specific parts of animal brains describing the effects on movement and behavior.[246]
Modern period
Studies of the brain became more sophisticated with the use of the microscope and the development of a silver staining method by Camillo Golgi during the 1880s. This was able to show the intricate structures of single neurons.[247] This was used by Santiago Ramón y Cajal and led to the formation of the neuron doctrine, the then revolutionary hypothesis that the neuron is the functional unit of the brain. He used microscopy to uncover many cell types, and proposed functions for the cells he saw.[247] For this, Golgi and Cajal are considered the founders of twentieth century neuroscience, both sharing the Nobel prize in 1906 for their studies and discoveries in this field.[247]
Charles Sherrington published his influential 1906 work The Integrative Action of the Nervous System examining the function of reflexes, evolutionary development of the nervous system, functional specialisation of the brain, and layout and cellular function of the central nervous system.[248] In 1942 he coined the term enchanted loom as a metaphor for the brain. John Farquhar Fulton, founded the Journal of Neurophysiology and published the first comprehensive textbook on the physiology of the nervous system during 1938.[249] Neuroscience during the twentieth century began to be recognised as a distinct unified academic discipline, with David Rioch, Francis O. Schmitt, and Stephen Kuffler playing critical roles in establishing the field.[250] Rioch originated the integration of basic anatomical and physiological research with clinical psychiatry at the Walter Reed Army Institute of Research, starting in the 1950s.[251] During the same period, Schmitt established the Neuroscience Research Program, an inter-university and international organisation, bringing together biology, medicine, psychological and behavioural sciences. The word neuroscience itself arises from this program.[252]
Paul Broca associated regions of the brain with specific functions, in particular language in Broca's area, following work on brain-damaged patients.[253] John Hughlings Jackson described the function of the motor cortex by watching the progression of epileptic seizures through the body. Carl Wernicke described a region associated with language comprehension and production. Korbinian Brodmann divided regions of the brain based on the appearance of cells.[253] By 1950, Sherrington, Papez, and MacLean had identified many of the brainstem and limbic system functions.[254][255] The capacity of the brain to re-organise and change with age, and a recognised critical development period, were attributed to neuroplasticity, pioneered by Margaret Kennard, who experimented on monkeys during the 1930-40s.[256]
Harvey Cushing (1869–1939) is recognised as the first proficient brain surgeon in the world.[257] In 1937, Walter Dandy began the practice of vascular neurosurgery by performing the first surgical clipping of an intracranial aneurysm.[258]
Comparative anatomy
The human brain has many properties that are common to all vertebrate brains.[259] Many of its features are common to all mammalian brains,[260] most notably a six-layered cerebral cortex and a set of associated structures,[261] including the hippocampus and amygdala.[262] The cortex is proportionally larger in humans than in many other mammals.[263] Humans have more association cortex, sensory and motor parts than smaller mammals such as the rat and the cat.[264]
As a primate brain, the human brain has a much larger cerebral cortex, in proportion to body size, than most mammals,[262] and a highly developed visual system.[265][266]
As a hominid brain, the human brain is substantially enlarged even in comparison to the brain of a typical monkey. The sequence of human evolution from Australopithecus (four million years ago) to Homo sapiens (modern humans) was marked by a steady increase in brain size.[267][268] As brain size increased, this altered the size and shape of the skull,[269] from about 600 cm3 in Homo habilis to an average of about 1520 cm3 in Homo neanderthalensis.[270] Differences in DNA, gene expression, and gene–environment interactions help explain the differences between the function of the human brain and other primates.[271]
See also
- Cerebral atrophy
- Cortical spreading depression
- Evolution of human intelligence
- Large-scale brain networks
- Outline of neuroscience
- Superficial veins of the brain
References
- ↑ "Encephalo- Etymology". Encephalo- Etymology. http://etymonline.com/index.php?allowed_in_frame=0&search=encephalo-. Retrieved October 24, 2015.
- ↑ Parent, A.; Carpenter, M.B. (1995). "Ch. 1". Carpenter's Human Neuroanatomy. Williams & Wilkins. ISBN 978-0-683-06752-1.
- ↑ 3.0 3.1 Bigos, K.L.; Hariri, A.; Weinberger, D. (2015). Neuroimaging Genetics: Principles and Practices. Oxford University Press. p. 157. ISBN 978-0-19-992022-8. https://books.google.com/books?id=TF_iCgAAQBAJ&pg=PA157.
- ↑ 4.0 4.1 Cosgrove, K.P.; Mazure, C.M.; Staley, J.K. (2007). "Evolving knowledge of sex differences in brain structure, function, and chemistry". Biol Psychiatry 62 (8): 847–855. doi:10.1016/j.biopsych.2007.03.001. PMID 17544382.
- ↑ Molina, D. Kimberley; DiMaio, Vincent J.M. (2012). "Normal Organ Weights in Men". The American Journal of Forensic Medicine and Pathology 33 (4): 368–372. doi:10.1097/PAF.0b013e31823d29ad. ISSN 0195-7910. PMID 22182984.
- ↑ Molina, D. Kimberley; DiMaio, Vincent J. M. (2015). "Normal Organ Weights in Women". The American Journal of Forensic Medicine and Pathology 36 (3): 182–187. doi:10.1097/PAF.0000000000000175. ISSN 0195-7910. PMID 26108038.
- ↑ 7.0 7.1 7.2 7.3 Gray's Anatomy 2008, pp. 227-9.
- ↑ 8.0 8.1 Gray's Anatomy 2008, pp. 335-7.
- ↑ 9.0 9.1 Ribas, G. C. (2010). "The cerebral sulci and gyri". Neurosurgical Focus 28 (2): 7. doi:10.3171/2009.11.FOCUS09245. PMID 20121437.
- ↑ Frigeri, T.; Paglioli, E.; De Oliveira, E.; Rhoton Jr, A. L. (2015). "Microsurgical anatomy of the central lobe". Journal of Neurosurgery 122 (3): 483–98. doi:10.3171/2014.11.JNS14315. PMID 25555079.
- ↑ Møllgård, Kjeld; Beinlich, Felix R. M.; Kusk, Peter; Miyakoshi, Leo M.; Delle, Christine; Plá, Virginia; Hauglund, Natalie L.; Esmail, Tina et al. (2023). "A mesothelium divides the subarachnoid space into functional compartments". Science 379 (6627): 84–88. doi:10.1126/science.adc8810. PMID 36603070. Bibcode: 2023Sci...379...84M. https://www.researchgate.net/publication/366903551.
- University of Rochester Medical Center (January 5, 2023). "Newly discovered anatomy shields and monitors brain" (in en). https://medicalxpress.com/news/2023-01-newly-anatomy-shields-brain.html.
- ↑ Purves 2012, p. 724.
- ↑ 13.0 13.1 Cipolla, M.J. (January 1, 2009). "Anatomy and Ultrastructure". The Cerebral Circulation. Morgan & Claypool Life Sciences. https://www.ncbi.nlm.nih.gov/books/NBK53086/#s2.2.
- ↑ "A Surgeon's-Eye View of the Brain". May 10, 2006. https://www.npr.org/templates/story/story.php?storyId=5396115.
- ↑ Sampaio-Baptista, C; Johansen-Berg, H (December 20, 2017). "White Matter Plasticity in the Adult Brain". Neuron 96 (6): 1239–1251. doi:10.1016/j.neuron.2017.11.026. PMID 29268094.
- ↑ Davey, G. (2011). Applied Psychology. John Wiley & Sons. p. 153. ISBN 978-1-4443-3121-9. https://books.google.com/books?id=K1qq1SsgoxUC&pg=PA153.
- ↑ Arsava, E. Y.; Arsava, E. M.; Oguz, K. K.; Topcuoglu, M. A. (2019). "Occipital petalia as a predictive imaging sign for transverse sinus dominance". Neurological Research 41 (4): 306–311. doi:10.1080/01616412.2018.1560643. PMID 30601110.
- ↑ 18.0 18.1 Ackerman, S. (1992). Discovering the brain. Washington, D.C.: National Academy Press. pp. 22–25. ISBN 978-0-309-04529-2. https://archive.org/details/discoveringbrain00acke.
- ↑ Larsen 2001, pp. 455–456.
- ↑ Kandel, E.R.; Schwartz, J.H.; Jessel T.M. (2000). Principles of Neural Science. McGraw-Hill Professional. p. 324. ISBN 978-0-8385-7701-1. https://archive.org/details/isbn_9780838577011/page/324.
- ↑ Gray's Anatomy 2008, pp. 227–9.
- ↑ Guyton & Hall 2011, p. 574.
- ↑ Guyton & Hall 2011, p. 667.
- ↑ Principles of Anatomy and Physiology 12th Edition – Tortora, p. 519.
- ↑ 25.0 25.1 25.2 Freberg, L. (2009). Discovering Biological Psychology. Cengage Learning. pp. 44–46. ISBN 978-0-547-17779-3. https://books.google.com/books?id=-zyTMXAjzQsC&pg=PA44.
- ↑ 26.0 26.1 Kolb, B.; Whishaw, I. (2009). Fundamentals of Human Neuropsychology. Macmillan. pp. 73–75. ISBN 978-0-7167-9586-5. https://books.google.com/books?id=z0DThNQqdL4C&pg=PA73.
- ↑ Pocock 2006, p. 64.
- ↑ 28.0 28.1 Purves 2012, p. 399.
- ↑ Gray's Anatomy 2008, pp. 325-6.
- ↑ Goll, Y.; Atlan, G.; Citri, A. (August 2015). "Attention: the claustrum". Trends in Neurosciences 38 (8): 486–95. doi:10.1016/j.tins.2015.05.006. PMID 26116988.
- ↑ Goard, M.; Dan, Y. (October 4, 2009). "Basal forebrain activation enhances cortical coding of natural scenes". Nature Neuroscience 12 (11): 1444–1449. doi:10.1038/nn.2402. PMID 19801988.
- ↑ Guyton & Hall 2011, p. 699.
- ↑ 33.0 33.1 33.2 Gray's Anatomy 2008, p. 298.
- ↑ Netter, F. (2014). Atlas of Human Anatomy Including Student Consult Interactive Ancillaries and Guides. (6th ed.). Philadelphia, Penn.: W B Saunders Co. p. 114. ISBN 978-1-4557-0418-7.
- ↑ 35.0 35.1 Gray's Anatomy 2008, p. 297.
- ↑ Guyton & Hall 2011, pp. 698–9.
- ↑ Squire 2013, pp. 761–763.
- ↑ 38.0 38.1 38.2 38.3 38.4 38.5 Gray's Anatomy 2008, p. 275.
- ↑ Guyton & Hall 2011, p. 691.
- ↑ Purves 2012, p. 377.
- ↑ 41.0 41.1 Azevedo, F. (April 10, 2009). "Equal numbers of neuronal and nonneuronal cells make the human brain an isometrically scaled-up primate brain". The Journal of Comparative Neurology 513 (5): 532–541. doi:10.1002/cne.21974. PMID 19226510. "despite the widespread quotes that the human brain contains 100 billion neurons and ten times more glial cells, the absolute number of neurons and glial cells in the human brain remains unknown. Here we determine these numbers by using the isotropic fractionator and compare them with the expected values for a human-sized primate. We find that the adult male human brain contains on average 86.1 ± 8.1 billion NeuN-positive cells (“neurons”) and 84.6 ± 9.8 billion NeuN-negative (“nonneuronal”) cells.".
- ↑ Pavel, Fiala; Jiří, Valenta (January 1, 2013). Central Nervous System. Karolinum Press. p. 79. ISBN 978-80-246-2067-1. https://books.google.com/books?id=LPlSBAAAQBAJ&pg=PA79.
- ↑ 43.0 43.1 43.2 43.3 Polyzoidis, S.; Koletsa, T.; Panagiotidou, S.; Ashkan, K.; Theoharides, T.C. (2015). "Mast cells in meningiomas and brain inflammation". Journal of Neuroinflammation 12 (1): 170. doi:10.1186/s12974-015-0388-3. PMID 26377554.
- ↑ 44.0 44.1 44.2 44.3 44.4 Guyton & Hall 2011, pp. 748–749.
- ↑ Budzyński, J; Kłopocka, M. (2014). "Brain-gut axis in the pathogenesis of Helicobacter pylori infection". World J. Gastroenterol. 20 (18): 5212–25. doi:10.3748/wjg.v20.i18.5212. PMID 24833851.
- ↑ Carabotti, M.; Scirocco, A.; Maselli, M.A.; Severi, C. (2015). "The gut-brain axis: interactions between enteric microbiota, central and enteric nervous systems". Ann Gastroenterol 28 (2): 203–209. PMID 25830558.
- ↑ Sjöstedt, Evelina; Fagerberg, Linn; Hallström, Björn M.; Häggmark, Anna; Mitsios, Nicholas; Nilsson, Peter; Pontén, Fredrik; Hökfelt, Tomas et al. (June 15, 2015). "Defining the human brain proteome using transcriptomics and antibody-based profiling with a focus on the cerebral cortex". PLOS ONE 10 (6): e0130028. doi:10.1371/journal.pone.0130028. ISSN 1932-6203. PMID 26076492. Bibcode: 2015PLoSO..1030028S.
- ↑ 48.0 48.1 48.2 48.3 Gray's Anatomy 2008, pp. 242–244.
- ↑ Purves 2012, p. 742.
- ↑ Gray's Anatomy 2008, p. 243.
- ↑ Iliff, JJ; Nedergaard, M (June 2013). "Is there a cerebral lymphatic system?". Stroke 44 (6 Suppl 1): S93-5. doi:10.1161/STROKEAHA.112.678698. PMID 23709744.
- ↑ Gaillard, F.. "Glymphatic pathway". https://radiopaedia.org/articles/glymphatic-pathway.
- ↑ 53.0 53.1 53.2 "The Paravascular Pathway for Brain Waste Clearance: Current Understanding, Significance and Controversy". Frontiers in Neuroanatomy 11: 101. November 2017. doi:10.3389/fnana.2017.00101. PMID 29163074. "The paravascular pathway, also known as the “glymphatic” pathway, is a recently described system for waste clearance in the brain. According to this model, cerebrospinal fluid (CSF) enters the paravascular spaces surrounding penetrating arteries of the brain, mixes with interstitial fluid (ISF) and solutes in the parenchyma, and exits along paravascular spaces of draining veins. ... In addition to Aβ clearance, the glymphatic system may be involved in the removal of other interstitial solutes and metabolites. By measuring the lactate concentration in the brains and cervical lymph nodes of awake and sleeping mice, Lundgaard et al. (2017) demonstrated that lactate can exit the CNS via the paravascular pathway. Their analysis took advantage of the substantiated hypothesis that glymphatic function is promoted during sleep (Xie et al., 2013; Lee et al., 2015; Liu et al., 2017).".
- ↑ Dissing-Olesen, L.; Hong, S.; Stevens, B. (August 2015). "New brain lymphatic vessels drain old concepts". eBioMedicine 2 (8): 776–7. doi:10.1016/j.ebiom.2015.08.019. PMID 26425672.
- ↑ 55.0 55.1 Sun, BL; Wang, LH; Yang, T; Sun, JY; Mao, LL; Yang, MF; Yuan, H; Colvin, RA et al. (April 2018). "Lymphatic drainage system of the brain: A novel target for intervention of neurological diseases.". Progress in Neurobiology 163–164: 118–143. doi:10.1016/j.pneurobio.2017.08.007. PMID 28903061.
- ↑ Gray's Anatomy 2008, p. 247.
- ↑ Gray's Anatomy 2008, pp. 251-2.
- ↑ 58.0 58.1 58.2 Gray's Anatomy 2008, p. 250.
- ↑ 59.0 59.1 Gray's Anatomy 2008, p. 248.
- ↑ 60.0 60.1 Gray's Anatomy 2008, p. 251.
- ↑ 61.0 61.1 61.2 Gray's Anatomy 2008, pp. 254-6.
- ↑ 62.0 62.1 62.2 62.3 62.4 Elsevier's 2007, pp. 311–4.
- ↑ Daneman, R.; Zhou, L.; Kebede, A.A.; Barres, B.A. (November 25, 2010). "Pericytes are required for blood-brain barrier integrity during embryogenesis". Nature 468 (7323): 562–6. doi:10.1038/nature09513. PMID 20944625. Bibcode: 2010Natur.468..562D.
- ↑ Laterra, J. et al. (1999). Basic neurochemistry: molecular, cellular and medical aspects (6th ed.). Philadelphia: Lippincott-Raven.
- ↑ Sadler, T. (2010). Langman's medical embryology (11th ed.). Philadelphia: Lippincott Williams & Wilkins. p. 293. ISBN 978-0-7817-9069-7.
- ↑ 66.0 66.1 Larsen 2001, p. 419.
- ↑ Zhou, Yi; Song, Hongjun; Ming, Guo-Li (2023-07-28). "Genetics of human brain development". Nature Reviews. Genetics 25 (1): 26–45. doi:10.1038/s41576-023-00626-5. ISSN 1471-0064. PMID 37507490. https://pubmed.ncbi.nlm.nih.gov/37507490.
- ↑ 68.0 68.1 68.2 Larsen 2001, pp. 85–88.
- ↑ Purves 2012, pp. 480–482.
- ↑ 70.0 70.1 70.2 70.3 Larsen 2001, pp. 445–446.
- ↑ "OpenStax CNX". http://cnx.org/contents/b037bde2-ea37-43a5-9102-8d4fcbc623d1@3/The_Embryologic_Perspective.
- ↑ Larsen 2001, pp. 85–87.
- ↑ Purves 2012, pp. 481–484.
- ↑ Purves, Dale; Augustine, George J; Fitzpatrick, David et al., eds (2001). "Rhombomeres". Neuroscience (2nd ed.). Palgrave Macmillan. ISBN 978-0-87893-742-4. https://www.ncbi.nlm.nih.gov/books/NBK10954/box/A1478/.
- ↑ 75.0 75.1 Chen, X. (2012). Mechanical Self-Assembly: Science and Applications. Springer Science & Business Media. pp. 188–189. ISBN 978-1-4614-4562-3. https://books.google.com/books?id=94aPR_Oh40oC&pg=PA188.
- ↑ 76.0 76.1 76.2 Ronan, L; Voets, N; Rua, C; Alexander-Bloch, A; Hough, M; Mackay, C; Crow, TJ; James, A et al. (August 2014). "Differential tangential expansion as a mechanism for cortical gyrification.". Cerebral Cortex 24 (8): 2219–28. doi:10.1093/cercor/bht082. PMID 23542881.
- ↑ Van Essen, DC (January 23, 1997). "A tension-based theory of morphogenesis and compact wiring in the central nervous system.". Nature 385 (6614): 313–8. doi:10.1038/385313a0. PMID 9002514. Bibcode: 1997Natur.385..313E.
- ↑ Borrell, V (24 January 2018). "How Cells Fold the Cerebral Cortex.". The Journal of Neuroscience 38 (4): 776–783. doi:10.1523/JNEUROSCI.1106-17.2017. PMID 29367288.
- ↑ Florio, M. (March 27, 2015). "Human-specific gene ARHGAP11B promotes basal progenitor amplification and neocortex expansion". Science 347 (6229): 1465–70. doi:10.1126/science.aaa1975. PMID 25721503. Bibcode: 2015Sci...347.1465F.
- ↑ "Parts of the Brain | Introduction to Psychology". https://courses.lumenlearning.com/wmopen-psychology/chapter/outcome-parts-of-the-brain/.
- ↑ Guyton & Hall 2011, p. 685.
- ↑ 82.0 82.1 Guyton & Hall 2011, p. 687.
- ↑ 83.0 83.1 Guyton & Hall 2011, p. 686.
- ↑ Guyton & Hall 2011, pp. 698, 708.
- ↑ Davidson's 2010, p. 1139.
- ↑ 86.0 86.1 Hellier, J. (2014). The Brain, the Nervous System, and Their Diseases [3 volumes]. ABC-CLIO. pp. 300–303. ISBN 978-1-61069-338-7. https://books.google.com/books?id=SDi2BQAAQBAJ&pg=PA300.
- ↑ 87.0 87.1 Guyton & Hall 2011, pp. 571–576.
- ↑ Guyton & Hall 2011, pp. 573–574.
- ↑ Guyton & Hall 2011, pp. 623-631.
- ↑ Guyton & Hall 2011, pp. 739–740.
- ↑ Pocock 2006, pp. 138–139.
- ↑ Squire 2013, pp. 525–526.
- ↑ Guyton & Hall 2011, pp. 647–648.
- ↑ Guyton & Hall 2011, pp. 202–203.
- ↑ Guyton & Hall 2011, pp. 205–208.
- ↑ 96.0 96.1 96.2 96.3 Guyton & Hall 2011, pp. 505–509.
- ↑ "Brain Basics: Understanding Sleep | National Institute of Neurological Disorders and Stroke". https://www.ninds.nih.gov/Disorders/Patient-Caregiver-Education/Understanding-Sleep.
- ↑ Guyton & Hall 2011, p. 723.
- ↑ Davis, J.F.; Choi, D.L.; Benoit, S.C. (2011). "24. Orexigenic Hypothalamic Peptides Behavior and Feeding – 24.5 Orexin". in Preedy, V.R.; Watson, R.R.; Martin, C.R.. Handbook of Behavior, Food and Nutrition. Springer. pp. 361–362. ISBN 978-0-387-92271-3. https://books.google.com/books?id=KuAEPOPbW6MC&pg=PA361.
- ↑ Squire 2013, p. 800.
- ↑ Squire 2013, p. 803.
- ↑ Squire 2013, p. 805.
- ↑ Guyton & Hall 2011, pp. 720-2.
- ↑ Poeppel, D.; Emmorey, K.; Hickok, G.; Pylkkänen, L. (October 10, 2012). "Towards a new neurobiology of language". The Journal of Neuroscience 32 (41): 14125–14131. doi:10.1523/JNEUROSCI.3244-12.2012. PMID 23055482.
- ↑ Hickok, G (September 2009). "The functional neuroanatomy of language". Physics of Life Reviews 6 (3): 121–143. doi:10.1016/j.plrev.2009.06.001. PMID 20161054. Bibcode: 2009PhLRv...6..121H.
- ↑ Fedorenko, E.; Kanwisher, N. (2009). "Neuroimaging of language: why hasn't a clearer picture emerged?". Language and Linguistics Compass 3 (4): 839–865. doi:10.1111/j.1749-818x.2009.00143.x.
- ↑ Damasio, H. (2001). "Neural basis of language disorders". in Chapey, Roberta. Language intervention strategies in aphasia and related neurogenic communication disorders (4th ed.). Lippincott Williams & Wilkins. pp. 18–36. ISBN 978-0-7817-2133-2. OCLC 45952164.
- ↑ de Lussanet, M.H.E.; Osse, J.W.M. (2012). "An ancestral axial twist explains the contralateral forebain and the optic chiasm in vertebrates". Animal Biology 62 (2): 193–216. doi:10.1163/157075611X617102.
- ↑ Hellier, J. (2014). The Brain, the Nervous System, and Their Diseases [3 volumes]. ABC-CLIO. p. 1135. ISBN 978-1-61069-338-7. https://books.google.com/books?id=SDi2BQAAQBAJ&pg=PA1135.
- ↑ Kolb, B.; Whishaw, I.Q. (2013). Introduction to Brain and Behavior. Macmillan Higher Education. p. 296. ISBN 978-1-4641-3960-4. https://books.google.com/books?id=teUkAAAAQBAJ.
- ↑ Berntson, G.; Cacioppo, J. (2009). Handbook of Neuroscience for the Behavioral Sciences, Volume 1. John Wiley & Sons. p. 145. ISBN 978-0-470-08355-0. https://books.google.com/books?id=LwdJhh8bOvwC&pg=PA145.
- ↑ Sherwood, L. (2012). Human Physiology: From Cells to Systems. Cengage Learning. p. 181. ISBN 978-1-133-70853-7. https://books.google.com/books?id=CZkJAAAAQBAJ&pg=PT181.
- ↑ Kalat, J (2015). Biological Psychology. Cengage Learning. p. 425. ISBN 978-1-305-46529-9. https://books.google.com/books?id=EzZBBAAAQBAJ&pg=PA425.
- ↑ 114.0 114.1 Cowin, S.C.; Doty, S.B. (2007). Tissue Mechanics. Springer Science & Business Media. p. 4. ISBN 978-0-387-49985-7. https://books.google.com/books?id=8BJhRkat--YC&pg=PA4.
- ↑ 115.0 115.1 Morris, C.G.; Maisto, A.A. (2011). Understanding Psychology. Prentice Hall. p. 56. ISBN 978-0-205-76906-3. https://books.google.com/books?id=hoVWAAAAYAAJ.
- ↑ 116.0 116.1 Kolb, B.; Whishaw, I.Q. (2013). Introduction to Brain and Behavior (Loose-Leaf). Macmillan Higher Education. pp. 524–549. ISBN 978-1-4641-3960-4. https://books.google.com/books?id=teUkAAAAQBAJ.
- ↑ Schacter, D.L.; Gilbert, D.T.; Wegner, D.M. (2009). Introducing Psychology. Macmillan. p. 80. ISBN 978-1-4292-1821-4. https://books.google.com/books?id=gt8lpZylVmkC&pg=PA80.
- ↑ Sander, David (2013). Armony, J.; Vuilleumier, Patrik. eds. The Cambridge handbook of human affective neuroscience. Cambridge: Cambridge Univ. Press. p. 16. ISBN 978-0-521-17155-7.
- ↑ Lindquist, KA.; Wager, TD.; Kober, H; Bliss-Moreau, E; Barrett, LF (May 23, 2012). "The brain basis of emotion: A meta-analytic review". Behavioral and Brain Sciences 35 (3): 121–143. doi:10.1017/S0140525X11000446. PMID 22617651.
- ↑ Phan, KL; Wager, Tor; Taylor, SF.; Liberzon, l (June 1, 2002). "Functional Neuroanatomy of Emotion: A Meta-Analysis of Emotion Activation Studies in PET and fMRI". NeuroImage 16 (2): 331–348. doi:10.1006/nimg.2002.1087. PMID 12030820.
- ↑ Malenka, RC; Nestler, EJ; Hyman, SE (2009). "Preface". in Sydor, A; Brown, RY. Molecular Neuropharmacology: A Foundation for Clinical Neuroscience (2nd ed.). New York: McGraw-Hill Medical. p. xiii. ISBN 978-0-07-148127-4.
- ↑ 122.0 122.1 122.2 122.3 "Chapter 14: Higher Cognitive Function and Behavioral Control". Molecular Neuropharmacology: A Foundation for Clinical Neuroscience (3rd ed.). New York: McGraw-Hill Medical. 2015. ISBN 978-0-07-182770-6.
- ↑ 123.0 123.1 "Chapter 6: Widely Projecting Systems: Monoamines, Acetylcholine, and Orexin". Molecular Neuropharmacology: A Foundation for Clinical Neuroscience (3rd ed.). New York: McGraw-Hill Medical. 2015. ISBN 978-0-07-182770-6.
- ↑ 124.0 124.1 124.2 124.3 124.4 Diamond, A (2013). "Executive functions". Annual Review of Psychology 64: 135–168. doi:10.1146/annurev-psych-113011-143750. PMID 23020641.
Figure 4: Executive functions and related terms - ↑ 125.0 125.1 125.2 125.3 Hyun, J.C.; Weyandt, L.L.; Swentosky, A. (2014). "Chapter 2: The Physiology of Executive Functioning". in Goldstein, S.; Naglieri, J.. Handbook of Executive Functioning. New York: Springer. pp. 13–23. ISBN 978-1-4614-8106-5. https://books.google.com/books?id=1e8VAgAAQBAJ&pg=PA13.
- ↑ 126.0 126.1 "Chapter 14: Higher Cognitive Function and Behavioral Control". Molecular Neuropharmacology: A Foundation for Clinical Neuroscience (3rd ed.). New York: McGraw-Hill Medical. 2015. ISBN 978-0-07-182770-6. "In conditions in which prepotent responses tend to dominate behavior, such as in drug addiction, where drug cues can elicit drug seeking (Chapter 16), or in attention deficit hyperactivity disorder (ADHD; described below), significant negative consequences can result. ... ADHD can be conceptualized as a disorder of executive function; specifically, ADHD is characterized by reduced ability to exert and maintain cognitive control of behavior. Compared with healthy individuals, those with ADHD have diminished ability to suppress inappropriate prepotent responses to stimuli (impaired response inhibition) and diminished ability to inhibit responses to irrelevant stimuli (impaired interference suppression). ... Functional neuroimaging in humans demonstrates activation of the prefrontal cortex and caudate nucleus (part of the dorsal striatum) in tasks that demand inhibitory control of behavior. ... Early results with structural MRI show a thinner cerebral cortex, across much of the cerebrum, in ADHD subjects compared with age-matched controls, including areas of [the] prefrontal cortex involved in working memory and attention."
- ↑ Pocock 2006, p. 68.
- ↑ Clark, B.D.; Goldberg, E.M.; Rudy, B. (December 2009). "Electrogenic tuning of the axon initial segment.". The Neuroscientist 15 (6): 651–68. doi:10.1177/1073858409341973. PMID 20007821.
- ↑ Pocock 2006, pp. 70–74.
- ↑ 130.0 130.1 "NIMH » Brain Basics". https://www.nimh.nih.gov/health/educational-resources/brain-basics/brain-basics.shtml.
- ↑ Purves, Dale (2011). Neuroscience (5. ed.). Sunderland, Mass.: Sinauer. p. 139. ISBN 978-0-87893-695-3.
- ↑ Swaminathan, N (April 29, 2008). "Why Does the Brain Need So Much Power?". Scientific American. http://www.scientificamerican.com/article/why-does-the-brain-need-s/.
- ↑ 133.0 133.1 "Four grams of glucose". American Journal of Physiology. Endocrinology and Metabolism 296 (1): E11–21. January 2009. doi:10.1152/ajpendo.90563.2008. PMID 18840763. "Four grams of glucose circulates in the blood of a person weighing 70 kg. This glucose is critical for normal function in many cell types. In accordance with the importance of these 4 g of glucose, a sophisticated control system is in place to maintain blood glucose constant. Our focus has been on the mechanisms by which the flux of glucose from liver to blood and from blood to skeletal muscle is regulated. ... The brain consumes ~60% of the blood glucose used in the sedentary, fasted person. ... The amount of glucose in the blood is preserved at the expense of glycogen reservoirs (Fig. 2). In postabsorptive humans, there are ~100 g of glycogen in the liver and ~400 g of glycogen in muscle. Carbohydrate oxidation by the working muscle can go up by ~10-fold with exercise, and yet after 1 h, blood glucose is maintained at ~4 g. ... It is now well established that both insulin and exercise cause translocation of GLUT4 to the plasma membrane. Except for the fundamental process of GLUT4 translocation, [muscle glucose uptake (MGU)] is controlled differently with exercise and insulin. Contraction-stimulated intracellular signaling (52, 80) and MGU (34, 75, 77, 88, 91, 98) are insulin independent. Moreover, the fate of glucose extracted from the blood is different in response to exercise and insulin (91, 105). For these reasons, barriers to glucose flux from blood to muscle must be defined independently for these two controllers of MGU.".
- ↑ Quistorff, B; Secher, N; Van Lieshout, J (July 24, 2008). "Lactate fuels the human brain during exercise". The FASEB Journal 22 (10): 3443–3449. doi:10.1096/fj.08-106104. PMID 18653766.
- ↑ Obel, L.F.; Müller, M.S.; Walls, A.B.; Sickmann, H.M.; Bak, L.K.; Waagepetersen, H.S.; Schousboe, A. (2012). "Brain glycogen-new perspectives on its metabolic function and regulation at the subcellular level.". Frontiers in Neuroenergetics 4: 3. doi:10.3389/fnene.2012.00003. PMID 22403540.
- ↑ Marin-Valencia, I. (February 2013). "Heptanoate as a neural fuel: energetic and neurotransmitter precursors in normal and glucose transporter I-deficient (G1D) brain.". Journal of Cerebral Blood Flow and Metabolism 33 (2): 175–82. doi:10.1038/jcbfm.2012.151. PMID 23072752.
- ↑ Tsuji, A. (2005). "Small molecular drug transfer across the blood-brain barrier via carrier-mediated transport systems". NeuroRx 2 (1): 54–62. doi:10.1602/neurorx.2.1.54. PMID 15717057. "Uptake of valproic acid was reduced in the presence of medium-chain fatty acids such as hexanoate, octanoate, and decanoate, but not propionate or butyrate, indicating that valproic acid is taken up into the brain via a transport system for medium-chain fatty acids, not short-chain fatty acids. ... Based on these reports, valproic acid is thought to be transported bidirectionally between blood and brain across the BBB via two distinct mechanisms, monocarboxylic acid-sensitive and medium-chain fatty acid-sensitive transporters, for efflux and uptake, respectively.".
- ↑ Vijay, N.; Morris, M.E. (2014). "Role of monocarboxylate transporters in drug delivery to the brain". Curr. Pharm. Des. 20 (10): 1487–98. doi:10.2174/13816128113199990462. PMID 23789956. "Monocarboxylate transporters (MCTs) are known to mediate the transport of short chain monocarboxylates such as lactate, pyruvate and butyrate. ... MCT1 and MCT4 have also been associated with the transport of short chain fatty acids such as acetate and formate which are then metabolized in the astrocytes [78].".
- ↑ Clark, D.D.; Sokoloff. L. (1999). Basic Neurochemistry: Molecular, Cellular and Medical Aspects. Philadelphia: Lippincott. pp. 637–670. ISBN 978-0-397-51820-3.
- ↑ Mrsulja, B.B. (2012). Pathophysiology of Cerebral Energy Metabolism. Springer Science & Business Media. pp. 2–3. ISBN 978-1-4684-3348-7. https://books.google.com/books?id=8yzvBwAAQBAJ&pg=PA2.
- ↑ Raichle, M.; Gusnard, DA (2002). "Appraising the brain's energy budget". Proc. Natl. Acad. Sci. U.S.A. 99 (16): 10237–10239. doi:10.1073/pnas.172399499. PMID 12149485. Bibcode: 2002PNAS...9910237R.
- ↑ Gianaros, Peter J.; Gray, Marcus A.; Onyewuenyi, Ikechukwu; Critchley, Hugo D. (2010). "Neuroimaging Methods in Behavioral Medicine". in Steptoe, A.. Handbook of Behavioral Medicine. Springer Science & Business Media. p. 770. doi:10.1007/978-0-387-09488-5_50. ISBN 978-0-387-09488-5. https://books.google.com/books?id=Si9TtI5AGIEC&pg=PA770.
- ↑ Kuzawa, C. W.; Chugani, H. T.; Grossman, L. I.; Lipovich, L.; Muzik, O.; Hof, P. R.; Wildman, D. E.; Sherwood, C. C. et al. (2014-09-09). "Metabolic costs and evolutionary implications of human brain development". Proceedings of the National Academy of Sciences 111 (36): 13010–13015. doi:10.1073/pnas.1323099111. ISSN 0027-8424. PMID 25157149. Bibcode: 2014PNAS..11113010K.
- ↑ "Brain may flush out toxins during sleep". National Institutes of Health. http://www.ninds.nih.gov/news_and_events/news_articles/pressrelease_brain_sleep_10182013.htm.
- ↑ "Sleep drives metabolite clearance from the adult brain". Science 342 (6156): 373–377. October 2013. doi:10.1126/science.1241224. PMID 24136970. Bibcode: 2013Sci...342..373X. "Thus, the restorative function of sleep may be a consequence of the enhanced removal of potentially neurotoxic waste products that accumulate in the awake central nervous system.".
- ↑ Tononi, Guilio; Cirelli, Chiara (August 2013). "Perchance to Prune". Scientific American 309 (2): 34–39. doi:10.1038/scientificamerican0813-34. PMID 23923204. Bibcode: 2013SciAm.309b..34T. https://pdfs.semanticscholar.org/6f9d/f7817534e55865bd1f6b7da6d2912bdbeaf3.pdf.
- ↑ 147.0 147.1 Van Essen, D.C. (October 2012). "The Human Connectome Project: A data acquisition perspective". NeuroImage 62 (4): 2222–2231. doi:10.1016/j.neuroimage.2012.02.018. PMID 22366334.
- ↑ Jones, E.G.; Mendell, L.M. (April 30, 1999). "Assessing the Decade of the Brain". Science 284 (5415): 739. doi:10.1126/science.284.5415.739. PMID 10336393. Bibcode: 1999Sci...284..739J.
- ↑ "A $4.5 Billion Price Tag for the BRAIN Initiative?". June 5, 2014. https://www.science.org/content/article/45-billion-price-tag-brain-initiative.
- ↑ Fan, Xue; Markram, Henry (2019-05-07). "A Brief History of Simulation Neuroscience". Frontiers in Neuroinformatics 13: 32. doi:10.3389/fninf.2019.00032. ISSN 1662-5196. PMID 31133838.
- ↑ Amtul, Z; Rahman, AU (February 2016). "Neural Plasticity and Memory: Is Memory Encoded in Hydrogen Bonding Patterns?". The Neuroscientist : a review journal bringing neurobiology, neurology and psychiatry 22 (1): 9-18. doi:10.1177/1073858414547934. PMID 25168338.
- ↑ Chatterjee, S; Bahl, E; Mukherjee, U; Walsh, EN; Shetty, MS; Yan, AL; Vanrobaeys, Y; Lederman, JD et al. (25 March 2022). "Endoplasmic reticulum chaperone genes encode effectors of long-term memory.". Science advances 8 (12): eabm6063. doi:10.1126/sciadv.abm6063. PMID 35319980.
- ↑ Towle, V.L. (January 1993). "The spatial location of EEG electrodes: locating the best-fitting sphere relative to cortical anatomy". Electroencephalography and Clinical Neurophysiology 86 (1): 1–6. doi:10.1016/0013-4694(93)90061-y. PMID 7678386.
- ↑ Purves 2012, pp. 632–633.
- ↑ Silverstein, J. (2012). "Mapping the Motor and Sensory Cortices: A Historical Look and a Current Case Study in Sensorimotor Localization and Direct Cortical Motor Stimulation". The Neurodiagnostic Journal 52 (1): 54–68. PMID 22558647. http://www.readperiodicals.com/201203/2662763741.html.
- ↑ Boraud, T. et al. (2002). "From single extracellular unit recording in experimental and human Parkinsonism to the development of a functional concept of the role played by the basal ganglia in motor control". Progress in Neurobiology 66 (4): 265–283. doi:10.1016/s0301-0082(01)00033-8. PMID 11960681.
- ↑ Lancaster, MA; Renner, M; Martin, CA; Wenzel, D; Bicknell, LS; Hurles, ME; Homfray, T; Penninger, JM et al. (September 19, 2013). "Cerebral organoids model human brain development and microcephaly.". Nature 501 (7467): 373–9. doi:10.1038/nature12517. PMID 23995685. Bibcode: 2013Natur.501..373L.
- ↑ Lee, CT; Bendriem, RM; Wu, WW; Shen, RF (August 20, 2017). "3D brain Organoids derived from pluripotent stem cells: promising experimental models for brain development and neurodegenerative disorders.". Journal of Biomedical Science 24 (1): 59. doi:10.1186/s12929-017-0362-8. PMID 28822354.
- ↑ "Magnetic Resonance, a critical peer-reviewed introduction; functional MRI". European Magnetic Resonance Forum. http://www.magnetic-resonance.org/ch/11-03.html.
- ↑ Buxton, R.; Uludag, K.; Liu, T. (2004). "Modeling the haemodynamic response to brain activation". NeuroImage 23: S220–S233. doi:10.1016/j.neuroimage.2004.07.013. PMID 15501093.
- ↑ Biswal, B.B. (August 15, 2012). "Resting state fMRI: a personal history". NeuroImage 62 (2): 938–44. doi:10.1016/j.neuroimage.2012.01.090. PMID 22326802.
- ↑ Purves 2012, p. 20.
- ↑ Kane, R.L.; Parsons, T.D. (2017). The Role of Technology in Clinical Neuropsychology. Oxford University Press. p. 399. ISBN 978-0-19-023473-7. https://books.google.com/books?id=iuAwDgAAQBAJ. "Irimia, Chambers, Torgerson, and Van Horn (2012) provide a first-step graphic on how best to display connectivity findings, as is presented in Figure 13.15. This is referred to as a connectogram."
- ↑ Andrews, D.G. (2001). Neuropsychology. Psychology Press. ISBN 978-1-84169-103-9. https://books.google.com/books?id=kiCtU8wBTfwC.
- ↑ Lepage, M. (2010). "Research at the Brain Imaging Centre". Douglas Mental Health University Institute. http://www.douglas.qc.ca/page/imagerie-cerebrale?locale=en.
- ↑ 166.0 166.1 Steward, C.A. (2017). "Genome annotation for clinical genomic diagnostics: strengths and weaknesses". Genome Med 9 (1): 49. doi:10.1186/s13073-017-0441-1. PMID 28558813.
- ↑ Harrow, J. (September 2012). "GENCODE: the reference human genome annotation for The ENCODE Project.". Genome Res. 22 (9): 1760–74. doi:10.1101/gr.135350.111. PMID 22955987.
- ↑ A primer of genome science (3rd ed.). Sunderland, MA: Sinauer Associates. April 20, 2009. ISBN 9780878932368.
- ↑ "The human proteome in brain – The Human Protein Atlas". https://www.proteinatlas.org/humanproteome/brain.
- ↑ Uhlén, Mathias; Fagerberg, Linn; Hallström, Björn M.; Lindskog, Cecilia; Oksvold, Per; Mardinoglu, Adil; Sivertsson, Åsa; Kampf, Caroline et al. (January 23, 2015). "Tissue-based map of the human proteome". Science 347 (6220): 1260419. doi:10.1126/science.1260419. ISSN 0036-8075. PMID 25613900.
- ↑ Warden, A (2017). "Gene expression profiling in the human alcoholic brain.". Neuropharmacology 122: 161–174. doi:10.1016/j.neuropharm.2017.02.017. PMID 28254370.
- ↑ Farris, S.P. (2015). "Applying the new genomics to alcohol dependence.". Alcohol 49 (8): 825–36. doi:10.1016/j.alcohol.2015.03.001. PMID 25896098.
- ↑ Rozycka, A; Liguz-Lecznar, M (August 2017). "The space where aging acts: focus on the GABAergic synapse.". Aging Cell 16 (4): 634–643. doi:10.1111/acel.12605. PMID 28497576.
- ↑ Flores, CE; Méndez, P (2014). "Shaping inhibition: activity dependent structural plasticity of GABAergic synapses.". Frontiers in Cellular Neuroscience 8: 327. doi:10.3389/fncel.2014.00327. PMID 25386117.
- ↑ "Brain Injury, Traumatic". Medcyclopaedia. GE. http://www.medcyclopaedia.com/library/topics/volume_vi_1/b/BRAIN_INJURY_TRAUMATIC.aspx.
- ↑ Dawodu, S.T. (March 9, 2017). Traumatic Brain Injury (TBI) – Definition and Pathophysiology: Overview, Epidemiology, Primary Injury. http://emedicine.medscape.com/article/326510-overview#a3.
- ↑ Davidson's 2010, pp. 1196-7.
- ↑ "Shared proteomic effects of cerebral atherosclerosis and Alzheimer's disease on the human brain". Nat Neurosci 23 (6): 696–700. June 2020. doi:10.1038/s41593-020-0635-5. PMID 32424284.
- ↑ 179.0 179.1 Davidson's 2010, pp. 1205-15.
- ↑ 180.0 180.1 180.2 180.3 180.4 Davidson's 2010, pp. 1216-7.
- ↑ Volkow, N.D.; Koob, G.F.; McLellan, A.T. (January 2016). "Neurobiologic advances from the brain disease model of addiction". The New England Journal of Medicine 374 (4): 363–371. doi:10.1056/NEJMra1511480. PMID 26816013.
- ↑ Simpson, J.M.; Moriarty, G.L. (2013). Multimodal Treatment of Acute Psychiatric Illness: A Guide for Hospital Diversion. Columbia University Press. pp. 22–24. ISBN 978-0-231-53609-7. https://books.google.com/books?id=MbtkAgAAQBAJ&pg=PA22.
- ↑ 183.0 183.1 183.2 183.3 Davidson's 2010, pp. 1172-9.
- ↑ "Status Epilepticus". https://www.epilepsy.com/learn/challenges-epilepsy/seizure-emergencies/status-epilepticus.
- ↑ Moore, S.P. (2005). The Definitive Neurological Surgery Board Review. Lippincott Williams & Wilkins. p. 112. ISBN 978-1-4051-0459-3. https://books.google.com/books?id=mkK1a4mEx3IC&pg=PA112.
- ↑ Pennington, B.F. (2008). Diagnosing Learning Disorders, Second Edition: A Neuropsychological Framework. Guilford Press. pp. 3–10. ISBN 978-1-60623-786-1. https://books.google.com/books?id=LVV10L62z6kC&pg=PA3.
- ↑ Govaert, P.; de Vries, L.S. (2010). An Atlas of Neonatal Brain Sonography: (CDM 182–183). John Wiley & Sons. pp. 89–92. ISBN 978-1-898683-56-8. https://books.google.com/books?id=FzcaxpvV1JUC&pg=PA89.
- ↑ 188.0 188.1 Perese, E.F. (2012). Psychiatric Advanced Practice Nursing: A Biopsychsocial Foundation for Practice. F.A. Davis. pp. 82–88. ISBN 978-0-8036-2999-8. https://books.google.com/books?id=6X_2AAAAQBAJ&pg=PA82.
- ↑ Kearney, C.; Trull, T.J. (2016). Abnormal Psychology and Life: A Dimensional Approach. Cengage Learning. p. 395. ISBN 978-1-337-09810-6. https://books.google.com/books?id=B9q5DQAAQBAJ&pg=PA395.
- ↑ Stevenson, D.K.; Sunshine, P.; Benitz, W.E. (2003). Fetal and Neonatal Brain Injury: Mechanisms, Management and the Risks of Practice. Cambridge University Press. p. 191. ISBN 978-0-521-80691-6. https://books.google.com/books?id=RuekFAj_tIAC&pg=PA191.
- ↑ Dewhurst, John (2012). Dewhurst's Textbook of Obstetrics and Gynaecology. John Wiley & Sons. p. 43. ISBN 978-0-470-65457-6. https://books.google.com/books?id=HfakBRceodcC&pg=PA43.
- ↑ "Arteriovenous Malformations (AVMs) | National Institute of Neurological Disorders and Stroke". https://www.ninds.nih.gov/health-information/disorders/arteriovenous-malformations-avms?search-term=arteriovenous%20mal.
- ↑ Harbison, J.; Massey, A.; Barnett, L.; Hodge, D.; Ford, G.A. (June 1999). "Rapid ambulance protocol for acute stroke". Lancet 353 (9168): 1935. doi:10.1016/S0140-6736(99)00966-6. PMID 10371574.
- ↑ Davidson's 2010, p. 1183.
- ↑ 195.0 195.1 Davidson's 2010, pp. 1180-1.
- ↑ Davidson's 2010, pp. 1181, 1183-1185.
- ↑ 197.0 197.1 197.2 197.3 197.4 197.5 Davidson's 2010, pp. 1183-1185.
- ↑ 198.0 198.1 Davidson's 2010, pp. 1185-1189.
- ↑ Goyal, M. (April 2016). "Endovascular thrombectomy after large-vessel ischaemic stroke: a meta-analysis of individual patient data from five randomised trials". The Lancet 387 (10029): 1723–1731. doi:10.1016/S0140-6736(16)00163-X. PMID 26898852.
- ↑ Saver, J. L. (December 8, 2005). "Time is brain—quantified". Stroke 37 (1): 263–266. doi:10.1161/01.STR.0000196957.55928.ab. PMID 16339467.
- ↑ Winstein, C.J. (June 2016). "Guidelines for adult stroke rehabilitation and recovery". Stroke 47 (6): e98–e169. doi:10.1161/STR.0000000000000098. PMID 27145936.
- ↑ Kuźma, Elżbieta; Lourida, Ilianna; Moore, Sarah F.; Levine, Deborah A.; Ukoumunne, Obioha C.; Llewellyn, David J. (November 2018). "Stroke and dementia risk: A systematic review and meta-analysis". Alzheimer's & Dementia 14 (11): 1416–1426. doi:10.1016/j.jalz.2018.06.3061. ISSN 1552-5260. PMID 30177276.
- ↑ 203.0 203.1 203.2 203.3 Goila, AK; Pawar, M (2009). "The diagnosis of brain death". Indian Journal of Critical Care Medicine 13 (1): 7–11. doi:10.4103/0972-5229.53108. PMID 19881172.
- ↑ 204.0 204.1 204.2 Wijdicks, EFM (January 8, 2002). "Brain death worldwide: accepted fact but no global consensus in diagnostic criteria". Neurology 58 (1): 20–25. doi:10.1212/wnl.58.1.20. PMID 11781400.
- ↑ Dhanwate, AD (September 2014). "Brainstem death: A comprehensive review in Indian perspective.". Indian Journal of Critical Care Medicine 18 (9): 596–605. doi:10.4103/0972-5229.140151. PMID 25249744.
- ↑ 206.0 206.1 206.2 206.3 Davidson's 2010, p. 1158.
- ↑ Davidson's 2010, p. 200.
- ↑ Urden, L.D.; Stacy, K.M.; Lough, M.E. (2013). Priorities in Critical Care Nursing – E-Book. Elsevier Health Sciences. pp. 112–113. ISBN 978-0-323-29414-0. https://books.google.com/books?id=lLvwAwAAQBAJ&pg=PA112.
- ↑ Domínguez, J.F.; Lewis, E.D.; Turner, R.; Egan, G.F. (2009). Chiao, J.Y.. ed. "The Brain in Culture and Culture in the Brain: A Review of Core Issues in Neuroanthropology". Progress in Brain Research. Special issue: Cultural Neuroscience: Cultural Influences on Brain Function 178: 43–6. doi:10.1016/S0079-6123(09)17804-4. ISBN 978-0-444-53361-6. PMID 19874961.
- ↑ "Cultural Environment Influences Brain Function | Psych Central News". August 4, 2010. https://psychcentral.com/news/2010/08/04/cultural-environment-influences-brain-function/16380.html.
- ↑ 211.0 211.1 Macmillan, Malcolm B. (2000). An Odd Kind of Fame: Stories of Phineas Gage. MIT Press. ISBN 978-0-262-13363-0. https://books.google.com/books?id=Qx4fMsTqGFYC.
- ↑ Rescher, N. (1992). G. W. Leibniz's Monadology. Psychology Press. p. 83. ISBN 978-0-415-07284-7.
- ↑ Hart, WD (1996). Guttenplan S. ed. A Companion to the Philosophy of Mind. Blackwell. pp. 265–267.
- ↑ Churchland, P.S. (1989). "Ch. 8". Neurophilosophy. MIT Press. ISBN 978-0-262-53085-9. https://books.google.com/books?id=hAeFMFW3rDUC.
- ↑ Selimbeyoglu, Aslihan; Parvizi, J (2010). "Electrical stimulation of the human brain: perceptual and behavioral phenomena reported in the old and new literature". Frontiers in Human Neuroscience 4: 46. doi:10.3389/fnhum.2010.00046. PMID 20577584.
- ↑ Schwartz, J.H. Appendix D: Consciousness and the Neurobiology of the Twenty-First Century. In Kandel, E.R.; Schwartz, J.H.; Jessell, T.M. (2000). Principles of Neural Science, 4th Edition.
- ↑ Lilienfeld, S.O.; Lynn, S.J.; Ruscio, J.; Beyerstein, B.L. (2011). 50 Great Myths of Popular Psychology: Shattering Widespread Misconceptions about Human Behavior. John Wiley & Sons. p. 89. ISBN 978-1-4443-6074-5. https://books.google.com/books?id=8DlS0gfO_QUC&pg=PT89.
- ↑ McDaniel, M. (2005). "Big-brained people are smarter". Intelligence 33 (4): 337–346. doi:10.1016/j.intell.2004.11.005. http://www.people.vcu.edu/~mamcdani/Big-Brained%20article.pdf.
- ↑ Luders, E. (September 2008). "Mapping the relationship between cortical convolution and intelligence: effects of gender". Cerebral Cortex 18 (9): 2019–26. doi:10.1093/cercor/bhm227. PMID 18089578.
- ↑ Hoppe, C; Stojanovic, J (2008). "High-Aptitude Minds". Scientific American Mind 19 (4): 60–67. doi:10.1038/scientificamericanmind0808-60.
- ↑ "Tupaia belangeri". The Genome Institute, Washington University. http://genome.wustl.edu/genomes/view/tupaia_belangeri.
- ↑ Carrier, Martin; Mittelstrass, Jürgen (1991). Mind, Brain, Behavior: The Mind-body Problem and the Philosophy of Psychology (revised and expanded English ed.). Berlin: Walter de Gruyter. p. 11. ISBN 9783110128765. https://books.google.com/books?id=i7b7KgzRbJQC. Retrieved 22 May 2021. "[...] the Aristotelian view that the soul resides primarily in the heart [...]."
- ↑ Cobb, Matthew (April 21, 2020). The Idea of the Brain: The Past and Future of Neuroscience. New York: Hachette UK (published 2020). ISBN 9781541646865. https://books.google.com/books?id=VVmqDwAAQBAJ. Retrieved 22 May 2021. "[...] the ways in which we think about [the brain] are much richer than in the past, not simply because of the amazing facts we have discovered, but above all because of how we interpret them."
- ↑ Jarrett, C. (November 17, 2014). Great Myths of the Brain. John Wiley & Sons. ISBN 978-1-118-31271-1. https://books.google.com/books?id=fBPyBQAAQBAJ.
- ↑ Phillips, Helen (July 11, 2002). "Video game "brain damage" claim criticised". New Scientist. https://www.newscientist.com/article/dn2538-video-game-brain-damage-claim-criticised.html. Retrieved February 6, 2008.
- ↑ Popova, Maria (August 18, 2011). "'Brain Culture': How Neuroscience Became a Pop Culture Fixation". The Atlantic. https://www.theatlantic.com/health/archive/2011/08/brain-culture-how-neuroscience-became-a-pop-culture-fixation/243810/.
- ↑ Thornton, Davi Johnson (2011). Brain Culture. Neuroscience and Popular Media. Rutgers University Press. ISBN 978-0-8135-5013-8.
- ↑ Cyborgs and Space , in Astronautics (September 1960), by Manfred E. Clynes and Nathan S. Kline.
- ↑ Bergfelder, Tim (2005). International Adventures: German Popular Cinema and European Co-productions in the 1960s. Berghahn Books. p. 129. ISBN 978-1-57181-538-5. https://books.google.com/books?id=B1Nj41yxvZkC&pg=PA129.
- ↑ Kandel, ER; Schwartz JH; Jessell TM (2000). Principles of Neural Science (4th ed.). New York: McGraw-Hill. ISBN 978-0-8385-7701-1.
- ↑ 231.0 231.1 231.2 231.3 Gross, Charles G. (1987). Adelman, George. ed. Encyclopedia of neuroscience (2. ed.). Boston: Birkhäeuser. pp. 843–847. ISBN 978-0-8176-3335-6. http://www.princeton.edu/~cggross/Hist_Neurosci_Ency_neurosci.pdf.
- ↑ 232.0 232.1 Bear, M.F.; B.W. Connors; M.A. Paradiso (2001). Neuroscience: Exploring the Brain. Baltimore: Lippincott. ISBN 978-0-7817-3944-3.
- ↑ von Staden, p.157
- ↑ Swanson, Larry W. (August 12, 2014). Neuroanatomical Terminology: A Lexicon of Classical Origins and Historical Foundations. Oxford University Press. ISBN 978-0-19-534062-4. https://books.google.com/books?id=--PRAwAAQBAJ&q=nervous+system+anatomy+stagnation+galen+to+vesalius&pg=PA7.
- ↑ 235.0 235.1 Lokhorst, Gert-Jan (January 1, 2016). "Descartes and the Pineal Gland". Metaphysics Research Lab, Stanford University. https://plato.stanford.edu/entries/pineal-gland/.
- ↑ 236.0 236.1 236.2 236.3 236.4 236.5 Gross, Charles G. (1999). Brain, vision, memory : tales in the history of neuroscience. (1st MIT Press pbk. ed.). Cambridge, Mass.: MIT. pp. 37–51. ISBN 978-0-262-57135-7.
- ↑ Marshall, Louise H.; Magoun, Horace W. (March 9, 2013). Discoveries in the Human Brain: Neuroscience Prehistory, Brain Structure, and Function. Springer Science & Business Media. p. 44. ISBN 978-1-475-74997-7. https://books.google.com/books?id=guncBwAAQBAJ&q=vesalius&pg=PR5.
- ↑ Holtz, Anders; Levi, Richard (July 20, 2010). Spinal Cord Injury. Oxford University Press. ISBN 978-0-19-970681-5. https://books.google.com/books?id=ZvCqdwWwGRsC&pg=PA5.
- ↑ Tessman, Patrick A.; Suarez, Jose I. (2002). "Influence of early printmaking on the development of neuroanatomy and neurology". Archives of Neurology 59 (12): 1964–1969. doi:10.1001/archneur.59.12.1964. PMID 12470188.
- ↑ O'Connor, James (2003). "Thomas Willis and the background to Cerebri Anatome". Journal of the Royal Society of Medicine 96 (3): 139–143. doi:10.1177/014107680309600311. PMID 12612118.
- ↑ EMERY, ALAN (October 2000). "A Short History of Neurology: The British Contribution 1660–1910. Edited by F. CLIFFORD ROSE. (Pp. 282; illustrated; £25 Paperback; ISBN 07506 4165 7.) Oxford: Butterworth-Heinemann". Journal of Anatomy 197 (3): 513–518. doi:10.1046/j.1469-7580.2000.197305131.x.
- ↑ Olesko, Kathryn M.; Holmes, Frederic L. (1994). Cahan, David. ed. Experiment, Quantification, and Discovery: Helmholtz's Early Physiological Researches, 1843-50. Hermann von Helmholtz and the Foundations of Nineteenth Century Science. Berkeley; Los Angeles; London: University of California Press. pp. 50–108.
- ↑ Sabbatini, Renato M.E.. "Sabbatini, R.M.E.: The Discovery of Bioelectricity. Nerve Conduction". http://www.cerebromente.org.br/n06/historia/bioelectr3_i.htm.
- ↑ Finkelstein, Gabriel Ward (2013). Emil du Bois-Reymond : neuroscience, self, and society in nineteenth-century Germany. Cambridge, Massachusetts. ISBN 978-1-4619-5032-5. OCLC 864592470. https://www.worldcat.org/oclc/864592470.
- ↑ Karbowski, Kazimierz (February 14, 2008). "Sixty Years of Clinical Electroencephalography". European Neurology 30 (3): 170–175. doi:10.1159/000117338. PMID 2192889.
- ↑ Pearce, J.M.S. (March 17, 2009). "Marie-Jean-Pierre Flourens (1794–1867) and Cortical Localization". European Neurology 61 (5): 311–314. doi:10.1159/000206858. PMID 19295220.
- ↑ 247.0 247.1 247.2 De Carlos, Juan A.; Borrell, José (August 2007). "A historical reflection of the contributions of Cajal and Golgi to the foundations of neuroscience". Brain Research Reviews 55 (1): 8–16. doi:10.1016/j.brainresrev.2007.03.010. PMID 17490748.
- ↑ Burke, R.E. (April 2007). "Sir Charles Sherrington's The integrative action of the nervous system: a centenary appreciation". Brain 130 (Pt 4): 887–894. doi:10.1093/brain/awm022. PMID 17438014.
- ↑ Squire, Larry R., ed (1996). The history of neuroscience in autobiography. Washington DC: Society for Neuroscience. pp. 475–97. ISBN 978-0-12-660305-7.
- ↑ Cowan, W.M.; Harter, D.H.; Kandel, E.R. (2000). "The emergence of modern neuroscience: Some implications for neurology and psychiatry". Annual Review of Neuroscience 23: 345–346. doi:10.1146/annurev.neuro.23.1.343. PMID 10845068.
- ↑ Brady, Joseph V.; Nauta, Walle J. H. (October 22, 2013). Principles, Practices, and Positions in Neuropsychiatric Research: Proceedings of a Conference Held in June 1970 at the Walter Reed Army Institute of Research, Washington, D.C., in Tribute to Dr. David Mckenzie Rioch upon His Retirement as Director of the Neuropsychiatry Division of That Institute. Elsevier. p. vii. ISBN 978-1-4831-5453-4. https://books.google.com/books?id=AK4aAwAAQBAJ&pg=PR7.
- ↑ Adelman, George (January 15, 2010). "The Neurosciences Research Program at MIT and the Beginning of the Modern Field of Neuroscience". Journal of the History of the Neurosciences 19 (1): 15–23. doi:10.1080/09647040902720651. PMID 20391098.
- ↑ 253.0 253.1 Principles of Neural Science, 4th ed. Eric R. Kandel, James H. Schwartz, Thomas M. Jessel, eds. McGraw-Hill:New York, NY. 2000.
- ↑ Papez, J.W. (February 1995). "A proposed mechanism of emotion. 1937.". The Journal of Neuropsychiatry and Clinical Neurosciences 7 (1): 103–12. doi:10.1176/jnp.7.1.103. PMID 7711480.
- ↑ Lambert, Kelly G. (August 2003). "The life and career of Paul MacLean". Physiology & Behavior 79 (3): 343–349. doi:10.1016/S0031-9384(03)00147-1. PMID 12954429.
- ↑ Chatterjee, Anjan; Coslett, H. Branch (December 2013). The Roots of Cognitive Neuroscience: Behavioral Neurology and Neuropsychology. OUP USA. pp. 337–8. ISBN 978-0-19-539554-9. https://books.google.com/books?id=f9dMAgAAQBAJ&q=neuroscience+20th+century&pg=PA338.
- ↑ Bliss, Michael (October 1, 2005). Harvey Cushing : A Life in Surgery: A Life in Surgery. USA: Oxford University Press. pp. ix–x. ISBN 978-0-19-534695-4. https://books.google.com/books?id=EzbjVnjwjPYC.
- ↑ Kretzer, RM; Coon, AL; Tamargo, RJ (June 2010). "Walter E. Dandy's contributions to vascular neurosurgery". Journal of Neurosurgery 112 (6): 1182–91. doi:10.3171/2009.7.JNS09737. PMID 20515365.
- ↑ Glees, Paul (2005). The Human Brain. Cambridge University Press. p. 1. ISBN 978-0-521-01781-7. https://books.google.com/books?id=kWgeOPGdl_MC&pg=PA1.
- ↑ Simpkins, C. Alexander; Simpkins, Annellen M. (2012). Neuroscience for Clinicians: Evidence, Models, and Practice. Springer Science & Business Media. p. 143. ISBN 978-1-4614-4842-6. https://books.google.com/books?id=QG4LC-d2sm8C&pg=PA143.
- ↑ Bornstein, Marc H.; Lamb, Michael E. (2015). Developmental Science: An Advanced Textbook. Psychology Press. p. 220. ISBN 978-1-136-28220-1. https://books.google.com/books?id=XhA-CgAAQBAJ&pg=PA220.
- ↑ 262.0 262.1 Bernstein, Douglas (2010). Essentials of Psychology. Cengage Learning. p. 64. ISBN 978-0-495-90693-3. https://books.google.com/books?id=rd77N0KsLVkC&pg=PA64.
- ↑ Hofman, Michel A. (March 27, 2014). "Evolution of the human brain: when bigger is better". Frontiers in Neuroanatomy 8: 15. doi:10.3389/fnana.2014.00015. PMID 24723857.
- ↑ Gray, Peter (2002). Psychology (4th ed.). Worth Publishers. ISBN 978-0-7167-5162-5. OCLC 46640860. https://archive.org/details/psychology00gray.
- ↑ Lu, Zhong-Lin; Dosher, Barbara (2013). Visual Psychophysics: From Laboratory to Theory. MIT Press. p. 3. ISBN 978-0-262-01945-3. https://books.google.com/books?id=nYr6AQAAQBAJ&pg=PA3.
- ↑ Sharwood Smith, Mike (2017). Introducing Language and Cognition. Cambridge University Press. p. 206. ISBN 978-1-107-15289-2. https://books.google.com/books?id=fe-SDQAAQBAJ&pg=PA206.
- ↑ Kolb, Bryan; Whishaw, Ian Q. (2013). Introduction to Brain and Behavior. Macmillan Higher Education. p. 21. ISBN 978-1-4641-3960-4. https://books.google.com/books?id=teUkAAAAQBAJ.
- ↑ Nieuwenhuys, Rudolf; ten Donkelaar, Hans J.; Nicholson, Charles (2014). The Central Nervous System of Vertebrates. Springer. p. 2127. ISBN 978-3-642-18262-4. https://books.google.com/books?id=gsDqCAAAQBAJ&pg=PA2127.
- ↑ Lerner, Lee; Lerner, Brenda Wilmoth (2004). The Gale Encyclopedia of Science: Pheasants-Star. Gale. p. 3759. ISBN 978-0-7876-7559-2. https://books.google.com/books?id=mp7kcdK6SekC. "As human's position changed and the manner in which the skull balanced on the spinal column pivoted, the brain expanded, altering the shape of the cranium."
- ↑ Begun, David R. (2012). A Companion to Paleoanthropology. John Wiley & Sons. p. 388. ISBN 978-1-118-33237-5. https://books.google.com/books?id=oIoT1RcFeCwC&pg=PT388.
- ↑ Jones, R. (2012). "Neurogenetics: What makes a human brain?". Nature Reviews Neuroscience 13 (10): 655. doi:10.1038/nrn3355. PMID 22992645.
Bibliography
- Colledge, Nicki R.; Walker, Brian R.; Ralston, Stuart H. et al., eds (2010). Davidson's Principles and Practice of Medicine (21st ed.). Edinburgh: Churchill Livingstone/Elsevier. ISBN 978-0-7020-3085-7.
- Hall, John (2011). Guyton and Hall Textbook of Medical Physiology (12th ed.). Philadelphia, PA: Saunders/Elsevier. ISBN 978-1-4160-4574-8.
- Larsen, William J. (2001). Human Embryology (3rd ed.). Philadelphia, PA: Churchill Livingstone. ISBN 978-0-443-06583-5.
- Bogart, Bruce Ian; Ort, Victoria (2007). Elsevier's Integrated Anatomy and Embryology. Philadelphia, PA: Elsevier Saunders. ISBN 978-1-4160-3165-9.
- Pocock, G.; Richards, C. (2006). Human Physiology: The Basis of Medicine (3rd ed.). Oxford: Oxford University Press. ISBN 978-0-19-856878-0.
- Purves, Dale (2012). Neuroscience (5th ed.). Sunderland, MA: Sinauer associates. ISBN 978-0-87893-695-3.
- Squire, Larry (2013). Fundamental Neuroscience. Waltham, MA: Elsevier. ISBN 978-0-12-385870-2.
- Standring, Susan, ed (2008). Gray's Anatomy: The Anatomical Basis of Clinical Practice (40th ed.). London: Churchill Livingstone. ISBN 978-0-8089-2371-8.
Notes
- ↑ Specifically the oculomotor, trochlear nerve, trigeminal nerve, abducens nerve, facial nerve, vestibulocochlear nerve, glossopharyngeal nerve, vagus nerve, accessory nerve and hypoglossal nerves.[38]
- ↑ Including the vestibulo-ocular reflex, corneal reflex, gag reflex and dilation of the pupils in response to light,[206]
- ↑ Illustrated by architect Christopher Wren[236]
External links
- Brain facts and figures – Washington.edu
- Human brain – National Geographic
![]() | Original source: https://en.wikipedia.org/wiki/Human brain.
Read more |