Chemistry:Phosphorus mononitride
![]() | |||
| |||
Names | |||
---|---|---|---|
IUPAC name
Azanylidynephosphane
| |||
Other names
Phosphorus nitride
| |||
Identifiers | |||
3D model (JSmol)
|
|||
PubChem CID
|
|||
| |||
| |||
Properties | |||
PN | |||
Molar mass | 44.981 g/mol | ||
Except where otherwise noted, data are given for materials in their standard state (at 25 °C [77 °F], 100 kPa). | |||
Infobox references | |||
Phosphorus mononitride is an inorganic compound with the chemical formula PN. Containing only phosphorus and nitrogen, this material is classified as a binary nitride. From the Lewis structure perspective, it can be represented with a P-N triple bond with a lone pair on each atom. It is isoelectronic with N2, CO, P2, CS and SiO.
The compound is highly unstable in standard conditions, tending to rapidly self polymerize. It can be isolated within argon and krypton matrices at 10 K (−263.1 °C).[1] Due to its instability, documentation of reactions with other molecules is limited.[2] Most of its reactivity has thus far been probed and studied at transition metal centers. [3][4]
Phosphorus mononitride was the first identified phosphorus compound in the interstellar medium[5] and is even thought to be an important molecule in the atmospheres of Jupiter and Saturn.[6][7]
Discovery and interstellar occurrence
The existence of free, gas-phase phosphorus mononitride was confirmed spectroscopically in 1934 by Nobel laureate, Gerhard Herzberg, and coworkers.[8] J. Curry, L. Herzberg, and G. Herzberg made the accidental discovery after observing new bands in the UV region from 2375-2992 Å[9] following an electric discharge within an air-filled tube that had been earlier exposed to phosphorus.
In 1987, phosphorus mononitride was detected in the Orion KL Nebula, the W51M nebula in Aquila, and Saggitarius B2 simultaneously by Turner, Bally, and Ziurys. Data from radio telescopes allowed for observation of rotational lines associated with the J = 2-1, 3-2, 5-4, and 6-5 transitions.[5][10]
In the following decades, a rapid expansion of interstellar PN observations ensued, detected frequently alongside PO. Examples include within shocked regions of L1157,[11][12] within the galactic center,[13] in carbon-rich envelopes in CRL 2688 (alongside HCP) and oxygen-rich envelopes toward VY Canis Majoris,[14] TX Camelopardalis, R. Cassiopeiae, and NML Cygni.[15]
ALMA data alongside spectroscopic measurements from the Rosetta probe have shown PN being carried from the comet 67P/Churyumov–Gerasimenko alongside the far more abundant PO. [16] These observations may offer insight to how pre-biotic matter could be transported to planets. In cases where PN and PO are observed in the same region, the latter is more abundant. [17] The consistency of the molecular ratio between these two interstellar molecules across many different interstellar clouds is thought to be a sign of a shared formation pathway between the two molecules.[18] PN is mostly detected in hot, turbulent regions, where the shock induced sputtering of dust grain is thought to contribute to its formation. However, it has also been confirmed in massive dense cores which are by comparison "cold and quiescent".[19] [20]
In 2022, researchers used data from the ALMA Comprehensive High-resolution Extragalactic Molecular Inventory (ALCHEMI) project and reported evidence of phosphorus mononitride in giant molecular clouds within the galaxy, NGC 253. This finding marks phosphorus mononitride as the first extragalactic phosphorus containing molecule detected as well.[17] In 2023, Ziurys and coworkers showed the existence of PN and PO in WB89-621 (22.6 kpc from the galactic center) using rotational spectroscopy. Prior, phosphorus was only observed in the inner Milky Way (12kpc). Since supernovae do not occur in outer regions of the galaxy, the detection of these phosphorus-bearing molecules in WB89-621 provides evidence of additional alternative sources of phosphorus formation, such as non-explosive, lower mass asymptotic giant branch stars. The levels were detected at comparable values to that in the Solar system.[21]
Electronic structure, spectral and bonding properties
PN formation from gaseous phosphorus and nitrogen is endothermic.
½ P2 + ½ N2 = PN (ER = 117 ± 10kJ/mol)[25]
Early mass spectrometry studies by Gingerich yielded a PN dissociation energy D0 of 146.6 ± 5.0 kcal/mol (613 ± 21 kJ/mol; 6.36 ± 0.22 eV).[26]
It is predicted to have a high proton affinity (PA = 191 kcal/mol (800 kJ/mol)).[27]
Early rotational analysis of 24 of the bands from Herzberg's original study suggested a PN internuclear distance of 1.49 Å, intermediate between N2 (1.094 Å) and P2 (1.856 Å). The associated electronic transition, 1Π → 1Σ, was noted to be similar to that of the isoelectronic CS and SiO molecules.[28] Later rotational spectra studies aligned well with these findings, for example analysis of millimeter wave rotational PN spectra from a microwave spectrometer yielded a bond distance of 1.49085 (2) Å.[29][30]
Infrared studies of gaseous PN at high temperatures assign its vibrational frequency (ωe) to 1337.24 cm-1 and interatomic separation of 1.4869 Å. [8] [31]
Simple comparisons to tabulated experimental and calculated bond lengths match well with a PN triple bond according to Pyykkö’s Triple-Bond Covalent Radii.[32]
NBO analyses support a single neutral resonance structure with a PN triple bond and one lone pair on each atom. However, natural population analysis shows nitrogen as significantly negatively charged (-0.82603) and phosphorus as significantly positively charged (0.82603). This is in line with the large dipole moment and partial ionic character reflecting the electron density contour plots.[33][34][35][36][37][24]
Monomeric PN in a krypton matrix at 10 K (−263.1 °C) gives rise to a single IR band at 1323 cm-1.[1]
Auer and Neese have produced calculated gas phase 31P and 15N NMR chemical shifts of 51.61 and -344.71 respectively at the CCSD(T)/p4 level of theory.[38] However, different functionals and basis sets yield dramatically different predictions for chemical shielding and so far experimental NMR shifts for phosphorus mononitride remain elusive.[22]
Molecular beam electric resonance spectroscopy has been used to determine the radio frequency spectrum of phosphorus mononitride generated from P3N5 thermolysis; the experimental results showed an experimental PN dipole moment (μ) of 2.7465 +- 0.0001 D, 2.7380 +-0.001 D, and 2.7293 +-0.0001 D for the first three vibrational levels respectively.[39]
Its dipole moment is larger than PO (1.88 D), despite the greater electronegativity difference between the constituent P and O atoms and similar bond length (1.476 Å). [41] This a result of the significant differences in bonds and charge distribution within the PN and PO molecules. The large PN dipole moment makes it very favorable with respect to radio-astronomical studies in comparison to N2 - which lacks this property.[41]
In consideration to molecular orbitals of PN, direct analogies can be drawn to the bonding in the N2 molecule. It consists of an P-N σ bonding orbital (HOMO), with two perpendicular degenerate P-N pi bonding orbitals. Likewise, the LUMOs of PN, which consist of a degenerate PN pi-antibonding set, allow it to backbond with orbitals of appropriate symmetry.
However, in comparison to N2, the HOMO of PN is higher in energy (est. -9.2 eV vs -12.2 eV), and, the LUMOs are lower in energy (-2.3 eV vs -0.6 eV), thus making it both a better σ-donor and pi-acceptor as a ligand.[42]
Evidently, the smaller HOMO-LUMO gap of PN, combined with its polar nature and low dissociation energy contribute to its much greater reactivity than dinitrogen (including at the interstellar level).[43]
Preparation/Formation
Interstellar Formation
The pathways to the formation of PN are still not fully understood, but likely involve competing gaseous phase reactions with other interstellar molecules. Important schemes are shown below along with competing exothermic reactions:[44]
PO + N → PN + O
PO + N → P + NO (Competing)
Another important, very exothermic formation reaction:
PH + N → PN + H
From carbon containing environments:[45]
P + CN → PN + C
N + CP → PN + C
An important destruction pathway:
PN + N → N2 + P
The abundance of interstellar PN is additionally perturbed by cosmic-ray ionization, visual extinction, and adsorption/desorption from dust grains.[45][46]
Electric Discharge
Moldenhauer and Dörsam first generated transient PN in 1924 using an electric discharge through N2 and phosphorus vapors, where the characterized product was a notably robust powder containing equal parts phosphorus and nitrogen.[47] This same method led to the actual first observation of PN by Gerhard and coworkers.
PN has also been produced at room temperature using microwave discharges on mixtures of gaseous PCl3 and N2 under moderate vacuum. This preparation was employed to achieve high resolution FTIR spectra of PN.[48]
Flash Pyrolysis
Atkins and Timms later generated PN via flash pyrolysis of P3N5 under high vacuum, allowing the recording of the PN infrared spectrum within a cryogenic krypton matrix. Solid triphosphorus pentanitride generates gaseous, free PN when heated to 800–900 °C (1,070–1,170 K) under high vacuum.[1]

Monomeric PN can only be isolated in krypton or argon matrices at 10 K (−263.1 °C). Upon warming up past 30 K (−243.2 °C), cyclotriphosphazene, which has D3h symmetry, is formed (up to 50 K (−223.2 °C) before krypton matrix melts). The (PN)3 trimer and is planar and aromatic, with 15N-labelling experiments revealing a planar E' mode band at 1141 cm-1.[25] No dimers or other oligomers are even transiently observed.
Without a cryoscopic matrix, these reactions result in the immediate formation of (PN)n polymers.[1]
Thermolysis experiments of dimethyl phosphoramidate have shown PN to form as a major decomposition product along with many other minor components including the ·P=O radical and HOP=O. This is contrasting to dimethyl methylphosphonate in which said minor components become the major decomposition products, highlighting significantly diverging pathways.[49]

In 2023, Qian et al. proposed PN to be generated as a major product along with CO and cyclopentadienone byproducts when (o-phenyldioxyl)phosphinoazide is heated to 850 °C (following the loss of N2). However, efforts to observe free PN in argon matrixes using this method were unsuccessful due to band overlaps.[2]
Dehalogenation of Hexachlorophosphazene

Schnöckel and coworkers later showed an alternative synthesis involving the dehalogenation of hexachlorophosphazene with molten silver, with concomitant loss of AgCl. In both this route and the P3N5 thermolysis route, only trace P2 and P4 formation is detected even at 1,200 K (930 °C), showing the reaction temperatures occur far from thermodynamic equilibrium.[25]
Anthracene release from dibenzo-7λ3 -phosphanorbornadiene derivatives

The aforementioned methods require very high temperatures which are incompatible with standard, homogeneous solution state chemistry.
In 2022, Cummins and coworkers prepared and isolated a molecular PN precursor, N3PA which rapidly decomposes to N2, anthracene, and PN in solution at room temperature (t½ = 30 minutes). With the combination of vacuum and heating to 42 °C, this dissociation is explosive.[42]
Reactivity
Reactions of phosphorus mononitride with other molecules are rare and rather difficult to carry out. The formation of the intermediate (PN)3 trimer (which itself is only isolated in matrices) is highly favorable:
3PN ⇌ (PN)3 (-334 +/- 60 kJ/mol)[25]
PN generated in both the gaseous phase or in solution that is not subjected to trapping via noble gas matrices or particular metal complexes results in rapid self polymerization even in cases where trapping agents such as dienes or alkynes are present (differentiating its reactivity profile from related molecules such as P2).[50] [51]

Phosphorus mononitride's tendency to rapidly polymerize with itself has dominated its reactivity, greatly hindering both the study and diversity of products in its reactions with organic molecules.
In 2023, a rare case of documented reactivity with an organic molecule was reported by Qian and coworkers who demonstrated reversible photoisomerization between o-benzoquinone supported phosphinonitrene and o-benzoquinone stabilized phosphorus mononitride at 10 K, which can be isolated in an argon matrix.[2]
Ligation, Stabilization, and Reactivity at Transition Metals
The majority of documented well-defined PN reactivity has been carried out at transition metal centers. The electronic and molecular orbital similarities it shares with N2 make it a viable ligating species. While free PN is unstable, phosphorus mononitride has been prepared at metal coordination sites where it can exist as an isolable terminal ligand within a complex.[3][42] In alternative cases, PN ligands can also exist as only as transient, highly reactive intermediates featuring rich chemistry.[4] As a terminal ligand, cases of both preferential P and N bonding modes have been discovered.

Smith and co-workers isolated the first stable M-PN (and M-NP) complexes, using methodology to generate the PN moiety at metal sites. They reacted a tris(amido) Mo(VI) terminal phosphide complex with a tris(carbene)borate Fe(IV) terminal nitride, which undergo reductive coupling to form the corresponding neutral bridging PhB(iPr2Im)3Fe-NP-Mo(N3N) complex. Notably, the Mo-N-P bond angle in the bridging compound is nearly perfectly linear with an N-P bond length of 1.509(6) Å (only slightly elongated from free PN indicating significant multiple bond character).[3] Addition of 3 equivalents of strongly lewis basic tert-butyl isocyanide results in the release of the iron adduct as a [PhB(iPr2Im)3Fe-(CNtBu)3]+ cation in the second coordination sphere. The corresponding terminal linear Mo-PN anion can be isolated and converted to its linear Mo-NP isomer by exposure to white light in the solid state. The M-NP isomer of the ligand was determined to be more pi-acidic (N-P = 1.5913(1) Å and P-N = 1.5363(1) Å) and more thermodynamically stable than its isomer.[3]

Cummins and co-workers exploited their N3PA free PN releasing reagent to "trap" and isolate a stable terminal (dppe)(Cp*)Fe-NP complex as a BArF24 salt. The NP bond length in this case was very short at 1.493(2) Å, almost unperturbed from gaseous PN, which is consistent with minimal pi-backbonding from the iron center. Studies confirmed the NP binding mode (as opposed to PN) to be energetically preferred by 36.6 kcal/mol (153 kJ/mol) in this iron complex, creating a significant barrier to isomerization (thought to arise from Pauli repulsion effects).[42] Studies of phosphorus mononitride chemistry at tris(amido) vanadium complexes undertaken by Cummins and coworkers provides the bulk of PN reactivity examples at transition metals to date. In this system, PN is synthetically generated at a vanadium center from respective dibenzo-7λ3 -phosphanorbornadiene derivative precursors. However, it is not stable as a terminal ligand, and instead immediately undergoes trimerization. Notably, a thermodynamic equilibrium exists between this trimer species, along with a dimer and non-observed monomeric intermediate fragment.[4][50]
Fascinatingly, the V-NP fragment undergoes singlet phosphinidene reactivity ([2+1] additions) with alkene and alkyne trapping agents, generating phosphiranes and phospherenes respectively. The products generated from such additions exist in equilibrium (in the case with cis-4-octene and bis-trimethylsilylacetylene), where retention of the cis-4-octene conformer is observed. Upon heating, they reversibly add to generate the V-NP dimer. Such reactivity demonstrates stark contrasts from P2 as a ligand which instead undergoes formal cycloaddition chemistry.[4]
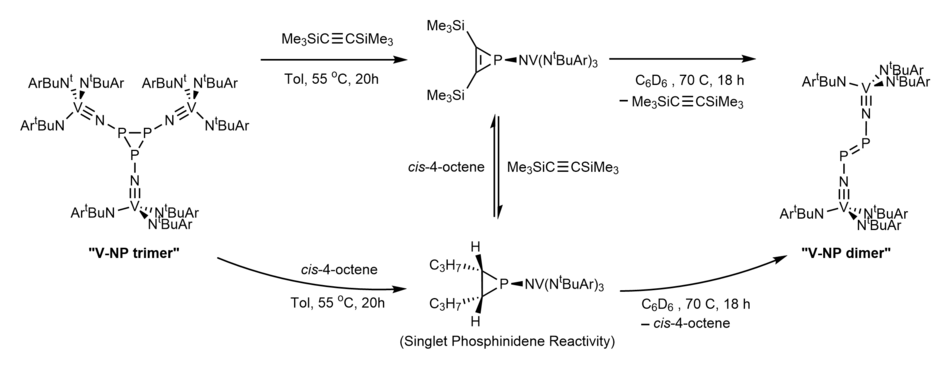

Other Relevant PN moieties
Bertrand and coworkers prepared an air stable PN group supported by neutral carbene NHC and CAAC ligands. However, the N-P bond length does not support significant multiple bond character as it features planar trans-bent geometry, with a P-N bond length of 1.7085 Å.[52]

In 1988, Niecke and co-workers provided the first example of a molecule containing a linear PN triple bond. The iminophosphenium tetrachloroaluminate salt was prepared from AlCl3 mediated chloride abstraction from the corresponding chloro(imino)phosphane, and features a very short bond length of 1.476 Å.[53]
Applications
The robust nature of PN reaction products such as (PN)n, could find use in heat resistant ceramics or as fire suppressing materials.[54]
There has long been interest in studying PN and its reaction products like (PN)n polymers, noting their relevance to precursors/intermediates in the production of fertilizers.[51] [55]
See also
References
- ↑ 1.0 1.1 1.2 1.3 1.4 Atkins, Robert M.; Timms, Peter L. (1977). "The matrix infrared spectrum of PN and SiS". Spectrochimica Acta Part A: Molecular Spectroscopy 33 (9): 853–857. doi:10.1016/0584-8539(77)80083-4. ISSN 0584-8539. Bibcode: 1977AcSpA..33..853A. http://dx.doi.org/10.1016/0584-8539(77)80083-4.
- ↑ 2.0 2.1 2.2 2.3 2.4 Qian, Weiyu; Wende, Raffael C.; Schreiner, Peter R.; Mardyukov, Artur (2023-04-18). "Selective Preparation of Phosphorus Mononitride (P≡N) from Phosphinoazide and Reversible Oxidation to Phosphinonitrene". Angewandte Chemie International Edition 62 (23): e202300761. doi:10.1002/anie.202300761. ISSN 1433-7851. PMID 36877095.
- ↑ 3.0 3.1 3.2 3.3 3.4 Martinez, Jorge L.; Lutz, Sean A.; Beagan, Daniel M.; Gao, Xinfeng; Pink, Maren; Chen, Chun-Hsing; Carta, Veronica; Moënne-Loccoz, Pierre et al. (2020-09-01). "Stabilization of the Dinitrogen Analogue, Phosphorus Nitride". ACS Central Science 6 (9): 1572–1577. doi:10.1021/acscentsci.0c00944. ISSN 2374-7943. PMID 32999932.
- ↑ 4.0 4.1 4.2 4.3 4.4 4.5 Courtemanche, Marc-André; Transue, Wesley J.; Cummins, Christopher C. (2016-12-21). "Phosphinidene Reactivity of a Transient Vanadium P≡N Complex" (in en). Journal of the American Chemical Society 138 (50): 16220–16223. doi:10.1021/jacs.6b10545. ISSN 0002-7863. PMID 27958729.
- ↑ 5.0 5.1 Turner, B. E.; Bally, John (1987-10-01). "Detection of Interstellar PN: The First Identified Phosphorus Compound in the Interstellar Medium". The Astrophysical Journal 321: L75. doi:10.1086/185009. ISSN 0004-637X. Bibcode: 1987ApJ...321L..75T. https://ui.adsabs.harvard.edu/abs/1987ApJ...321L..75T.
- ↑ Viana, Rommel B.; Pereira, Priscila S. S.; Macedo, Luiz G. M.; Pimentel, André S. (2009-09-18). "A quantum chemical study on the formation of phosphorus mononitride". Chemical Physics 363 (1): 49–58. doi:10.1016/j.chemphys.2009.07.008. ISSN 0301-0104. Bibcode: 2009CP....363...49V. https://www.sciencedirect.com/science/article/pii/S0301010409002353.
- ↑ Yung, Yuk L.; DeMore, William B. (1999-03-11), "Origins", Photochemistry of Planetary Atmospheres (Oxford University Press), doi:10.1093/oso/9780195105018.003.0007, ISBN 978-0-19-510501-8, http://dx.doi.org/10.1093/oso/9780195105018.003.0007, retrieved 2023-11-28
- ↑ 8.0 8.1 Curry, J.; Herzberg, L.; Herzberg, G. (1933-10-01). "Spectroscopic Evidence for the Molecule PN". The Journal of Chemical Physics 1 (10): 749. doi:10.1063/1.1749238. ISSN 0021-9606. Bibcode: 1933JChPh...1..749C. http://dx.doi.org/10.1063/1.1749238.
- ↑ Herzberg, G. (1972-07-14). "Spectroscopic Studies of Molecular Structure". Science 177 (4044): 123–138. doi:10.1126/science.177.4044.123. ISSN 0036-8075. PMID 17779905. Bibcode: 1972Sci...177..123H. http://dx.doi.org/10.1126/science.177.4044.123.
- ↑ Ziurys, L. M. (1987). "Detection of interstellar PN - The first phosphorus-bearing species observed in molecular clouds" (in en). The Astrophysical Journal 321 (1 Pt 2): L81-5. doi:10.1086/185010. ISSN 0004-637X. PMID 11542218. Bibcode: 1987ApJ...321L..81Z. http://adsabs.harvard.edu/doi/10.1086/185010.
- ↑ Yamaguchi, Takahiro; Takano, Shuro; Sakai, Nami; Sakai, Takeshi; Liu, Sheng-Yuan; Su, Yu-Nung; Hirano, Naomi; Takakuwa, Shigehisa et al. (2011-10-25). "Detection of Phosphorus Nitride in the Lynds 1157 B1 Shocked Region". Publications of the Astronomical Society of Japan 63 (5): L37–L41. doi:10.1093/pasj/63.5.l37. ISSN 0004-6264.
- ↑ Lefloch, Bertrand; Vastel, C.; Viti, S.; Jimenez-Serra, I.; Codella, C.; Podio, L.; Ceccarelli, C.; Mendoza, E. et al. (2016-08-02). "Phosphorus-bearing molecules in solar-type star-forming regions: first PO detection". Monthly Notices of the Royal Astronomical Society 462 (4): 3937–3944. doi:10.1093/mnras/stw1918. ISSN 0035-8711.
- ↑ Rivilla, V M; Jiménez-Serra, I; Zeng, S; Martín, S; Martín-Pintado, J; Armijos-Abendaño, J; Viti, S; Aladro, R et al. (2018-01-05). "Phosphorus-bearing molecules in the Galactic Center". Monthly Notices of the Royal Astronomical Society: Letters 475 (1): L30–L34. doi:10.1093/mnrasl/slx208. ISSN 1745-3925. http://dx.doi.org/10.1093/mnrasl/slx208.
- ↑ Milam, S. N.; Halfen, D. T.; Tenenbaum, E. D.; Apponi, A. J.; Woolf, N. J.; Ziurys, L. M. (2008). "Constraining Phosphorus Chemistry in Carbon- and Oxygen-Rich Circumstellar Envelopes: Observations of PN, HCP, and CP". The Astrophysical Journal 684 (1): 618–625. doi:10.1086/589135. ISSN 0004-637X. Bibcode: 2008ApJ...684..618M.
- ↑ Ziurys, L. M.; Schmidt, D. R.; Bernal, J. J. (2018-04-05). "New Circumstellar Sources of PO and PN: The Increasing Role of Phosphorus Chemistry in Oxygen-rich Stars". The Astrophysical Journal 856 (2): 169. doi:10.3847/1538-4357/aaafc6. ISSN 1538-4357. Bibcode: 2018ApJ...856..169Z.
- ↑ Rivilla, V M; Drozdovskaya, M N; Altwegg, K; Caselli, P; Beltrán, M T; Fontani, F; van der Tak, F F S; Cesaroni, R et al. (2020-01-15). "ALMA and ROSINA detections of phosphorus-bearing molecules: the interstellar thread between star-forming regions and comets". Monthly Notices of the Royal Astronomical Society 492 (1): 1180–1198. doi:10.1093/mnras/stz3336. ISSN 0035-8711.
- ↑ 17.0 17.1 Haasler, D.; Rivilla, V. M.; Martín, S.; Holdship, J.; Viti, S.; Harada, N.; Mangum, J.; Sakamoto, K. et al. (2022). "First extragalactic detection of a phosphorus-bearing molecule with ALCHEMI: Phosphorus nitride (PN)". Astronomy & Astrophysics 659: A158. doi:10.1051/0004-6361/202142032. ISSN 0004-6361. Bibcode: 2022A&A...659A.158H. http://dx.doi.org/10.1051/0004-6361/202142032.
- ↑ Bernal, J. J.; Koelemay, L. A.; Ziurys, L. M. (2021-01-01). "Detection of PO in Orion-KL: Phosphorus Chemistry in the Plateau Outflow". The Astrophysical Journal 906 (1): 55. doi:10.3847/1538-4357/abc87b. ISSN 0004-637X. Bibcode: 2021ApJ...906...55B.
- ↑ Fontani, F.; Rivilla, V. M.; Caselli, P.; Vasyunin, A.; Palau, A. (2016-05-06). "Phosphorus-Bearing Molecules in Massive Dense Cores". The Astrophysical Journal 822 (2): L30. doi:10.3847/2041-8205/822/2/L30. ISSN 2041-8213. Bibcode: 2016ApJ...822L..30F.
- ↑ Mininni, C; Fontani, F; Rivilla, V M; Beltrán, M T; Caselli, P; Vasyunin, A (2018-02-21). "On the origin of phosphorus nitride in star-forming regions". Monthly Notices of the Royal Astronomical Society: Letters 476 (1): L39–L44. doi:10.1093/mnrasl/sly026. ISSN 1745-3925. http://dx.doi.org/10.1093/mnrasl/sly026.
- ↑ Koelemay, L. A.; Gold, K. R.; Ziurys, L. M. (2023-11-08). "Phosphorus-bearing molecules PO and PN at the edge of the Galaxy". Nature 623 (7986): 292–295. doi:10.1038/s41586-023-06616-1. ISSN 0028-0836. PMID 37938703. Bibcode: 2023Natur.623..292K.
- ↑ 22.0 22.1 22.2 Kupka, Teobald; Leszczyńska, Małgorzata; Ejsmont, Krzysztof; Mnich, Adrianna; Broda, Małgorzata; Thangavel, Karthick; Kaminský, Jakub (2019-08-23). "Phosphorus mononitride: A difficult case for theory". International Journal of Quantum Chemistry 119 (24). doi:10.1002/qua.26032. ISSN 0020-7608. http://dx.doi.org/10.1002/qua.26032.
- ↑ 23.0 23.1 Lu, Tian; Chen, Feiwu (2011-12-08). "Multiwfn: A multifunctional wavefunction analyzer". Journal of Computational Chemistry 33 (5): 580–592. doi:10.1002/jcc.22885. ISSN 0192-8651. PMID 22162017. http://dx.doi.org/10.1002/jcc.22885.
- ↑ 24.0 24.1 24.2 Bader, Richard F W (1990-12-13). Atoms in Molecules. Oxford University PressOxford. doi:10.1093/oso/9780198551683.001.0001. ISBN 978-0-19-855168-3. http://dx.doi.org/10.1093/oso/9780198551683.001.0001.
- ↑ 25.0 25.1 25.2 25.3 25.4 Ahlrichs, Reinhart; Bär, Michael; Plitt, Harald S.; Schnöckel, Hansgeorg (1989). "The stability of PN and (PN)3. Ab initio calculations and matrix infrared investigations". Chemical Physics Letters 161 (2): 179–184. doi:10.1016/0009-2614(89)85053-5. ISSN 0009-2614. http://dx.doi.org/10.1016/0009-2614(89)85053-5.
- ↑ Gingerich, Karl A. (1969). "Gaseous phosphorus compounds. III. Mass spectrometric study of the reaction between diatomic nitrogen and phosphorus vapor and dissociation energy of phosphorus mononitride and diatomic phosphorus". The Journal of Physical Chemistry 73 (8): 2734–2741. doi:10.1021/j100842a047. ISSN 0022-3654. http://dx.doi.org/10.1021/j100842a047.
- ↑ Adams, N. G.; McIntosh, B. J.; Smith, D. (1990-06-01). "Production of phosphorus-containing molecules in interstellar clouds.". Astronomy and Astrophysics 232: 443. ISSN 0004-6361. Bibcode: 1990A&A...232..443A. https://ui.adsabs.harvard.edu/abs/1990A&A...232..443A.
- ↑ JEVONS, W. (1934). "Band Spectrum of PN and its Significance". Nature 133 (3364): 619–620. doi:10.1038/133619b0. ISSN 0028-0836. Bibcode: 1934Natur.133..619J.
- ↑ Hoeft, J.; Tiemann, E.; Törring, T. (1972-04-01). "Rotationsspektrum des PN". Zeitschrift für Naturforschung A 27 (4): 703–704. doi:10.1515/zna-1972-0424. ISSN 1865-7109. Bibcode: 1972ZNatA..27..703H.
- ↑ Wyse, F. C.; Manson, E. L.; Gordy, W. (1972-08-01). "Millimeter Wave Rotational Spectrum and Molecular Constants of 31P14N". The Journal of Chemical Physics 57 (3): 1106–1108. doi:10.1063/1.1678365. ISSN 0021-9606. http://dx.doi.org/10.1063/1.1678365.
- ↑ Maki, Arthur G.; Lovas, Frank J. (1981). "The infrared spectrum of 31P14N near 1300 cm−1". Journal of Molecular Spectroscopy 85 (2): 368–374. doi:10.1016/0022-2852(81)90209-5. ISSN 0022-2852. http://dx.doi.org/10.1016/0022-2852(81)90209-5.
- ↑ Pyykkö, Pekka; Atsumi, Michiko (2009-11-23). "Molecular Double-Bond Covalent Radii for Elements Li–E112" (in en). Chemistry – A European Journal 15 (46): 12770–12779. doi:10.1002/chem.200901472. ISSN 0947-6539. PMID 19856342. https://chemistry-europe.onlinelibrary.wiley.com/doi/10.1002/chem.200901472.
- ↑ Weinhold, F.; Landis, C.R.; Glendening, E.D. (2016-06-23). "What is NBO analysis and how is it useful?". International Reviews in Physical Chemistry 35 (3): 399–440. doi:10.1080/0144235x.2016.1192262. ISSN 0144-235X. http://dx.doi.org/10.1080/0144235x.2016.1192262.
- ↑ 34.0 34.1 34.2 Grimme, Stefan; Ehrlich, Stephan; Goerigk, Lars (2011). "Effect of the damping function in dispersion corrected density functional theory". Journal of Computational Chemistry 32 (7): 1456–1465. doi:10.1002/jcc.21759. ISSN 0192-8651. PMID 21370243. http://dx.doi.org/10.1002/jcc.21759.
- ↑ 35.0 35.1 35.2 Grimme, Stefan; Antony, Jens; Ehrlich, Stephan; Krieg, Helge (2010-04-16). "A consistent and accurate ab initio parametrization of density functional dispersion correction (DFT-D) for the 94 elements H-Pu". The Journal of Chemical Physics 132 (15). doi:10.1063/1.3382344. ISSN 0021-9606. PMID 20423165. Bibcode: 2010JChPh.132o4104G. http://dx.doi.org/10.1063/1.3382344.
- ↑ Weigend, Florian; Ahlrichs, Reinhart (2005). "Balanced basis sets of split valence, triple zeta valence and quadruple zeta valence quality for H to Rn: Design and assessment of accuracy". Physical Chemistry Chemical Physics 7 (18): 3297. doi:10.1039/b508541a. ISSN 1463-9076. PMID 16240044. Bibcode: 2005PCCP....7.3297W. http://dx.doi.org/10.1039/b508541a.
- ↑ Weigend, Florian (2006). "Accurate Coulomb-fitting basis sets for H to Rn". Physical Chemistry Chemical Physics 8 (9): 1057. doi:10.1039/b515623h. ISSN 1463-9076. Bibcode: 2006PCCP....8.1057W. http://dx.doi.org/10.1039/b515623h.
- ↑ Stoychev, Georgi L.; Auer, Alexander A.; Izsák, Róbert; Neese, Frank (2018-02-13). "Self-Consistent Field Calculation of Nuclear Magnetic Resonance Chemical Shielding Constants Using Gauge-Including Atomic Orbitals and Approximate Two-Electron Integrals" (in en). Journal of Chemical Theory and Computation 14 (2): 619–637. doi:10.1021/acs.jctc.7b01006. ISSN 1549-9618. PMID 29301077. https://pubs.acs.org/doi/10.1021/acs.jctc.7b01006.
- ↑ Raymonda, John; Klemperer, William (1971-07-01). "Molecular Beam Electric Resonance Spectrum of 31P14N". The Journal of Chemical Physics 55 (1): 232–233. doi:10.1063/1.1675513. ISSN 0021-9606. http://dx.doi.org/10.1063/1.1675513.
- ↑ 40.0 40.1 Knizia, Gerald (2013-10-17). "Intrinsic Atomic Orbitals: An Unbiased Bridge between Quantum Theory and Chemical Concepts". Journal of Chemical Theory and Computation 9 (11): 4834–4843. doi:10.1021/ct400687b. ISSN 1549-9618. PMID 26583402. http://dx.doi.org/10.1021/ct400687b.
- ↑ 41.0 41.1 Müller, Holger S. P.; Woon, David E. (2013-11-05). "Calculated Dipole Moments for Silicon and Phosphorus Compounds of Astrophysical Interest". The Journal of Physical Chemistry A 117 (50): 13868–13877. doi:10.1021/jp4083807. ISSN 1089-5639. PMID 24138156. Bibcode: 2013JPCA..11713868M. http://dx.doi.org/10.1021/jp4083807.
- ↑ 42.0 42.1 42.2 42.3 42.4 42.5 42.6 Eckhardt, André K.; Riu, Martin-Louis Y.; Ye, Mengshan; Müller, Peter; Bistoni, Giovanni; Cummins, Christopher C. (2022). "Taming phosphorus mononitride" (in en). Nature Chemistry 14 (8): 928–934. doi:10.1038/s41557-022-00958-5. ISSN 1755-4349. PMID 35697930. Bibcode: 2022NatCh..14..928E. https://www.nature.com/articles/s41557-022-00958-5.
- ↑ Bhasi, Priya; Nhlabatsi, Zanele P.; Sitha, Sanyasi (2017). "Reactivity of phosphorus mononitride and interstellar formation of molecules containing phospazo linkage: A computational study on the reaction between HSi (X2Π) and PN (X1Σ+)". Journal of Theoretical and Computational Chemistry 16 (8): 1750075. doi:10.1142/s0219633617500754. ISSN 0219-6336. http://dx.doi.org/10.1142/s0219633617500754.
- ↑ Millar, T. J.; Bennett, A.; Herbst, E. (1987-11-01). "An efficient gas phase synthesis for interstellar PN". Monthly Notices of the Royal Astronomical Society 229 (1): 41P–44P. doi:10.1093/mnras/229.1.41p. ISSN 0035-8711.
- ↑ 45.0 45.1 Chantzos, J.; Rivilla, V. M.; Vasyunin, A.; Redaelli, E.; Bizzocchi, L.; Fontani, F.; Caselli, P. (2020). "The first steps of interstellar phosphorus chemistry". Astronomy & Astrophysics 633: A54. doi:10.1051/0004-6361/201936531. ISSN 0004-6361. Bibcode: 2020A&A...633A..54C.
- ↑ Indriolo, Nick; McCall, Benjamin J. (2012-01-03). "INVESTIGATING THE COSMIC-RAY IONIZATION RATE IN THE GALACTIC DIFFUSE INTERSTELLAR MEDIUM THROUGH OBSERVATIONS OF H+3". The Astrophysical Journal 745 (1): 91. doi:10.1088/0004-637x/745/1/91. ISSN 0004-637X. Bibcode: 2012ApJ...745...91I.
- ↑ Moldenhauer, Wilhelm; Dörsam, H. (1926-05-05). "Über die Vereinigung von Phosphor und Stickstoff unter dem Einflusse elektrischer Entladungen". Berichte der Deutschen Chemischen Gesellschaft (A and B Series) 59 (5): 926–931. doi:10.1002/cber.19260590514. ISSN 0365-9488. http://dx.doi.org/10.1002/cber.19260590514.
- ↑ Ahmad, I.K.; Hamilton, P.A. (1995). "The Fourier Transform Infrared Spectrum of PN". Journal of Molecular Spectroscopy 169 (1): 286–291. doi:10.1006/jmsp.1995.1022. ISSN 0022-2852. Bibcode: 1995JMoSp.169..286A. http://dx.doi.org/10.1006/jmsp.1995.1022.
- ↑ Liang, Shuyu; Hemberger, Patrick; Levalois-Grützmacher, Joëlle; Grützmacher, Hansjörg; Gaan, Sabyasachi (2017-04-05). "Probing Phosphorus Nitride (P≡N) and Other Elusive Species Formed upon Pyrolysis of Dimethyl Phosphoramidate". Chemistry – A European Journal 23 (23): 5595–5601. doi:10.1002/chem.201700402. ISSN 0947-6539. PMID 28378378. http://dx.doi.org/10.1002/chem.201700402.
- ↑ 50.0 50.1 50.2 Eckhardt, André K.; Riu, Martin-Louis Y.; Müller, Peter; Cummins, Christopher C. (2022-01-12). "Staudinger Reactivity and Click Chemistry of Anthracene (A)-Based Azidophosphine N3PA". Inorganic Chemistry 61 (3): 1270–1274. doi:10.1021/acs.inorgchem.1c03753. ISSN 0020-1669. PMID 35020379. http://dx.doi.org/10.1021/acs.inorgchem.1c03753.
- ↑ 51.0 51.1 Huffman, E. O.; Tarbutton, Grady; Elmore, Kelly L.; Cate, W. E.; Walters, H. K.; Elmore, G. V. (1954). "Synthesis of Phosphorus Nitrides1". Journal of the American Chemical Society 76 (24): 6239–6243. doi:10.1021/ja01653a006. ISSN 0002-7863. http://dx.doi.org/10.1021/ja01653a006.
- ↑ 52.0 52.1 Kinjo, Rei; Donnadieu, Bruno; Bertrand, Guy (2010-08-09). "Isolation of a Carbene-Stabilized Phosphorus Mononitride and Its Radical Cation (PN +. )" (in en). Angewandte Chemie International Edition 49 (34): 5930–5933. doi:10.1002/anie.201002889. ISSN 1433-7851. https://onlinelibrary.wiley.com/doi/10.1002/anie.201002889.
- ↑ 53.0 53.1 Niecke, Edgar; Nieger, Martin; Reichert, Franz (1988). "Arylmino(halogeno)phosphanes XPNC 6 H 2 t Bu 3 (X Cl, Br, I) and the Iminophosphenium Tetrachloroaluminate [PNC 6 H 2 t Bu 3 ⊕ [AlCl 4 ] ⊖ : the First Stable Compound with a PN Triple Bond"] (in en). Angewandte Chemie International Edition in English 27 (12): 1715–1716. doi:10.1002/anie.198817151. ISSN 0570-0833. https://onlinelibrary.wiley.com/doi/10.1002/anie.198817151.
- ↑ Skaggs, S. R., Kaizerman, J., & Tapscott, R. E. (1995). Phosphorus Nitrides As Fire Extinguishing Agents. In Proceedings of Halon Options Technical Working Conference, Albuquerque, NM, USA (pp. 345-355).
- ↑ Hong, Ki Choong (1962). Synthesis of phosphorus nitride related high analysis fertilizers (Thesis). Iowa State University. doi:10.31274/rtd-180815-795.
NH3 | He(N2)11 | ||||||||||||||||
Li3N | Be3N2 | BN | β-C3N4 g-C3N4 |
N2 | NxOy | NF3 | Ne | ||||||||||
Na3N | Mg3N2 | AlN | Si3N4 | PN P3N5 |
SxNy SN S4N4 |
NCl3 | Ar | ||||||||||
K3N | Ca3N2 | ScN | TiN | VN | CrN Cr2N |
MnxNy | FexNy | CoN | Ni3N | CuN | Zn3N2 | GaN | Ge3N4 | As | Se | NBr3 | Kr |
Rb3N | Sr3N2 | YN | ZrN | NbN | β-Mo2N | Tc | Ru | Rh | PdN | Ag3N | CdN | InN | Sn | Sb | Te | NI3 | Xe |
Cs3N | Ba3N2 | Hf3N4 | TaN | WN | Re | Os | Ir | Pt | Au | Hg3N2 | TlN | Pb | BiN | Po | At | Rn | |
Fr3N | Ra3N | Rf | Db | Sg | Bh | Hs | Mt | Ds | Rg | Cn | Nh | Fl | Mc | Lv | Ts | Og | |
↓ | |||||||||||||||||
La | CeN | Pr | Nd | Pm | Sm | Eu | GdN | Tb | Dy | Ho | Er | Tm | Yb | Lu | |||
Ac | Th | Pa | UN | Np | Pu | Am | Cm | Bk | Cf | Es | Fm | Md | No | Lr |
![]() | Original source: https://en.wikipedia.org/wiki/Phosphorus mononitride.
Read more |